58 Andreas Neisius1 & Pei Zhong2 1 Department of Urology, Brüderkrankenhaus Trier, Johannes Gutenberg University, Mainz, Germany 2 Department of Mechanical Engineering and Materials Science, Duke University, Durham, NC, USA Since its inception in the early 1980s shock‐wave lithotripsy (SWL) has become the treatment of choice for the majority of urinary stones because of its non‐invasiveness compared to traditional stone treatments [1–3]. In the early 1990s more than 85% of stone patients in Europe and the United States were treated with SWL [4]. Although this trend has been in decline since then because of the rapid advance in endourological technology and technique, still about 70% of kidney stone patients in 2002 and fewer than 50% in 2005 were managed by SWL [2, 4–6]. A primary factor that has contributed to this gradual decline in the clinical use of SWL is the reduced treatment efficiency, compounded by the increased retreatment rate and higher risk of tissue injury produced by the second‐ and third‐generation lithotripters, compared to the first‐generation HM3 lithotripter [7, 8]. Although SWL technology has evolved significantly over the past two decades, there is still debate regarding the physical basis and clinical benefits of technical modifications such as the tight focal width and high peak pressure fields often used in many contemporary lithotripters [9–11]. Better design as well as effective and safe use of shock‐wave lithotripters must rely on a clear and fundamental understanding about the physics of this innovative technology. The physics and acoustical principles of SWL have been reviewed previously [12–14]. This chapter will therefore focus on the most salient features of SWL physics that are relevant to understanding the mechanisms of stone comminution and tissue injury with emphasis on contemporary technologies. The materials in this chapter are condensed in acoustics and physics content from a recent review of the topic by the senior author [15], but otherwise expanded based on the latest studies in order to provide a guideline for better lithotripter design, and effective and safe clinical use of this non‐invasive technology for stone management. The overarching goal of SWL is to pulverize kidney stones into gravels for spontaneous discharge in urine without inflicting adverse effects to surrounding tissues. As illustrated in Figure 58.1, disintegration of kidney stones by focused shock waves is a progressive process in which fragments of different size, shape, and distribution in the renal collecting system will be produced that may all influence the clinical outcome. In particular, the rate of stone comminution is not uniform throughout SWL. Stone disintegration accelerates initially within a few hundred shocks, followed by a gradually decelerating process towards the end of treatment. This critical observation suggests that different mechanisms of stone fragmentation may exist during the course of SWL, a unique feature that was not taken into account in the design of the second‐ and third‐generation lithotripters. To succeed in SWL, it is important to gain a fundamental understanding about acoustic waves (Box 58.1), the characteristics of focused shock waves produced by lithotripters (Box 58.2), and the physical basis for the optimal selection of lithotripter parameters and treatment protocol (or strategy) to maximize stone comminution while minimizing tissue injury. Figure 58.1 (a) Progressive comminution of a 10 mm spherical artificial stone in a membrane holder. (b) Dose‐dependence in stone comminution (solid lines) and normalized rate of stone comminution (dashed lines) produced by a contemporary electromagnetic shock‐wave lithotripter. Source: from [15] with permission of Springer‐Verlag, Berlin, Heidelberg. All clinical lithotripters are constructed using four primary components: (i) shock‐wave generator, (ii) focusing device, (iii) coupling medium, and (iv) stone localization system. The original Dornier HM3 lithotripter employed a water tub for coupling to ensure effective transmission of the lithotripter‐generated shock wave (LSW) without significant loss of energy at the patient’s surface (Figure 58.2a). In contrast, for operation convenience all contemporary lithotripters use dry coupling modalities with a water cushion system (such as the Siemens Lithoskop shown in Figure 58.2b). Three primary types of shock‐wave lithotripters have been developed – electrohydraulic (EH), electromagnetic (EM), and piezoelectric (PE) shock sources – with a variety of focusing systems (Figure 58.3). Stone localization in modern lithotripters is performed using isocentric C‐arm fluoroscopy with optional inline or offline ultrasound imaging [3]. The combination of the shock‐wave generator and the focusing device determines the main characteristics of the lithotripter field. Figure 58.2 Shock‐wave lithotripters: (a) first‐generation Dornier HM3 and (b) third‐generation Siemens Lithoskop. Source: from [15] with permission of Springer‐Verlag, Berlin, Heidelberg. Figure 58.3 Different types of shock‐wave lithotripters. EH, electrohydraulic; EM, electromagnetic; PE, piezoelectric. Source: from [15] with permission of Springer‐Verlag, Berlin, Heidelberg. In EH lithotripters, a spherically divergent shock wave is generated by a high voltage (12–30 kV) discharge between the tips of an electrode, which vaporizes and expands supersonically the surrounding fluid. In EM lithotripters, a magnetic field is produced by applying a high voltage (8–20 kV) pulse to a coil in flat, cylindrical, or spherical configuration to inject a strong current into the coil that repels an adjacent thin metallic membrane (in which a counter current is induced) to launch a high‐amplitude acoustic wave into the surrounding fluid. In PE lithotripters, a high‐voltage (1–10 kV) pulse is applied simultaneously to a mosaic of hundreds to few thousands piezoceramic elements that are mounted on a spherical carrier to generate a strong converging acoustic wave towards a common focus. Shock waves in EM and PE lithotripters are formed by nonlinear wave propagation in the coupling medium or interposing soft tissues between the shock source and the lithotripter focus [13]. Different designs of acoustic reflector and lens have been utilized for shock‐wave focusing. In EH lithotripters, the spark‐generated shock wave (at F1) is focused by a truncated ellipsoidal reflector to the second focus (F2) of the ellipsoid where the kidney stones are aligned under imaging guidance. In majority of EM lithotripters, either an acoustic lens or parabolic reflectors are used (Figure 58.3). In PE lithotripters, self‐focusing ensured by the geometrical configuration of the shock‐wave source is typically utilized. Self‐focusing is also employed in a recently developed EM lithotripter by Eisenmenger et al. [16]. Representative shock waves measured at the focus of the three different types of lithotripters are shown in Figure 58.4. A fiber‐optic probe hydrophone (FOPH) [17] recommended for lithotripter output characterization by the IEC standard is often used for such measurements [18]. All shock waves at the lithotripter focus consist of a leading shock front with a positive peak pressure (p+) of 40–70 MPa (1 MPa is about 10 times the atmospheric pressure) and an associated compressive wave of 1–2 microseconds in duration (1 microsecond = 1 millionth of a second). This fast rise of the compressive wave is referred to as “shock.” After falling to zero there is then a region of tensile wave lasting about 3–5 microseconds in duration with a peak negative pressure (p−) about −10 MPa. The entire shock‐wave pulse duration varies from 5 to 10 microseconds. Shock waves are broadband acoustic waves with a fundamental frequency in the range of 150–800 kHz (Figure 58.4a inset) [13]. As depicted in Figure 58.4a there is a notable pressure oscillation after the tensile wave produced by EM and PE lithotripters but not in EH lithotripters. In contrary to EH lithotripters in which the rise time of the leading shock front is less than 30 ns the shock‐wave rise time is about 100 ns for EM and PE lithotripters [13]. In general, pressure amplitudes are machine‐ and output‐setting‐specific, and the values of p + can vary from 20 to 120 MPa and p − from −4 to −15 MPa (see Table 58.1). Figure 58.4 (a) Pressure waveforms produced by different types of lithotripters and (b) model calculated peak pressure distribution in an EM lithotripter. EH, electrohydraulic; EM, electromagnetic; PE, piezoelectric. Source: from [15] with permission of Springer‐Verlag, Berlin, Heidelberg. Limited aperture size of the shock source causes the pressure field to be elongated along the central axis of the lithotripter to form a so‐called “cigar‐shaped” focus (Figure 58.4b). The size of the lithotripter focal region is measured by the −6 dB focal width (i.e., the dimension in which the positive pressure is equal or above half of p+) along the longitudinal and transverse axis of the lithotripter, respectively. It should be noted that the focal width is only a measure of the tightness of the pressure concentration in a lithotripter field. In general, the focal width may not be equated to the dimension of the fragmentation zone in a lithotripter field. To understand the mechanisms of stone fragmentation some basic features of kidney stones need to be considered. Kidney stones vary substantially in their chemical composition and structure. Stones are about 95% aggregated crystalline substances by weight, which are bound by cellular matrix proteins [28, 29]. As depicted in Figure 58.5 the composition of a kidney stone can often change from the core to the shell, as revealed by micro‐computed tomography (CT) images [30]. Calcium oxalate monohydrate (COM) or dihydrate (COD) stones represent the most common kidney stones. Other prevalent compositions include calcium hydrogen phosphate dihydrate (or brushite), carbonate‐apatite (CA), magnesium ammonium phosphate hexahydrate (MAPH or struvite), uric acid (UA), and cystine. It has been observed that the fragility of kidney stones during SWL varies significantly with composition and structural heterogeneity [31–33]. Figure 58.5 Example of the complexity of mineral regions revealed by micro‐computed tomography (CT). (a) Photomicrograph of stone on millimeter grid paper; (b) micro‐CT slice through the same stone. Minerals appear to be quite varied, as judged by morphology and X‐ray attenuation; e.g., there is a low‐attenuation region on the stone exterior (labeled 1) that is distinct from the high‐attenuation shell surrounding the stone (2). Beneath that shell are some regions that appear to be void of mineral (3), interspersed among crystalline extensions of an evenly absorbing material (4); inside there is a layer that absorbs X‐rays less well (5), which is in turn lined by a highly absorbing mineral (6); finally, the innermost region of the stone (7) is filled with a low‐absorbing material that could be subdivided into two or more morphologies. Source: from [30] with permission of Springer‐Verlag, Berlin, Heidelberg. Table 58.2 summarizes the acoustic and mechanical properties of kidney stones of different compositions, together with their counterpart values in artificial kidney stones that are often used in research to understand the mechanisms of stone fragmentation, and for comparisons of different lithotripters and treatment strategies. Table 58.2 Acoustic and physical properties of kidney stones of different chemical compositions and artificial stones. Source: from [15] with permission of Springer‐Verlag, Berlin, Heidelberg. Energy flux density ratio (IP/Ii and IS/Ii) for the refracted P and S waves in stones are their respective maximum values. COM, calcium oxalate monohydrate; UA, uric acid; CA, carbonate apatite; MAPH, magnesium ammonium phosphate hexahydrate; PoP, plaster of Paris; BS, BegoStone. θP*, critical incident of the pressure wave in the fluid that leads to total reflection of the P wave in the solid; θS*, critical incident of the pressure wave in the fluid that leads to total reflection of the S wave in the solid. E, Young’s modulus; G, shear modulus; HV, Vicker’s hardness; KIC, fracture toughness; σf, tensile failure strength of the solid. Stone fragmentation in SWL is the direct consequence of shock‐wave–stone interaction, which is illustrated in Figure 58.6 by high‐speed photoelastic and shadowgraph imaging [34]. When a LSW strikes on the stone surface, part of the wave will be reflected back into the water while the other part will be refracted (or transmitted) into the stone. In general, the wave refraction at a fluid/solid boundary will produce two types of stress wave in the solid. One is called the longitudinal (or P) wave, in which the solid particles vibrate in the same direction as the wave propagation. The other is called the transverse (or S) wave, in which the solid particles vibrate perpendicular to the direction of the wave propagation. The acoustical principles of wave reflection and refraction at fluid/solid boundaries are described in Box 58.3. In addition, cavitation – the formation, expansion, and collapse of gas/vapor bubbles in liquids – will be generated by the tensile component of the LSW, which plays an important, yet different, role in stone comminution and in tissue injury. Figure 58.6 High‐speed photoelastic and shadowgraph imaging sequence of shock‐wave–stone interaction and cavitation activities produced by an HM3 lithotripter in water around an artificial stone (an epoxy disk of 14 mm in diameter). DSW, direct shock wave; FSW, focused shock wave; SW, shock wave. P and S denote the longitudinal (or P) and transverse (or S) wavefronts in the epoxy sample. Time zero corresponds to the spark discharge. Source: from [15] with permission of Springer‐Verlag, Berlin, Heidelberg.
Physics of Shock‐wave Lithotripsy
Introduction
Overview of the clinical goal and physical process in SWL
General principles of shock‐wave lithotripsy
Shock‐wave generation
Shock‐wave focusing
Lithotripter‐generated shock‐wave profile and distribution
Composition and physical properties of kidney stones
Mechanisms of stone fragmentation in SWL
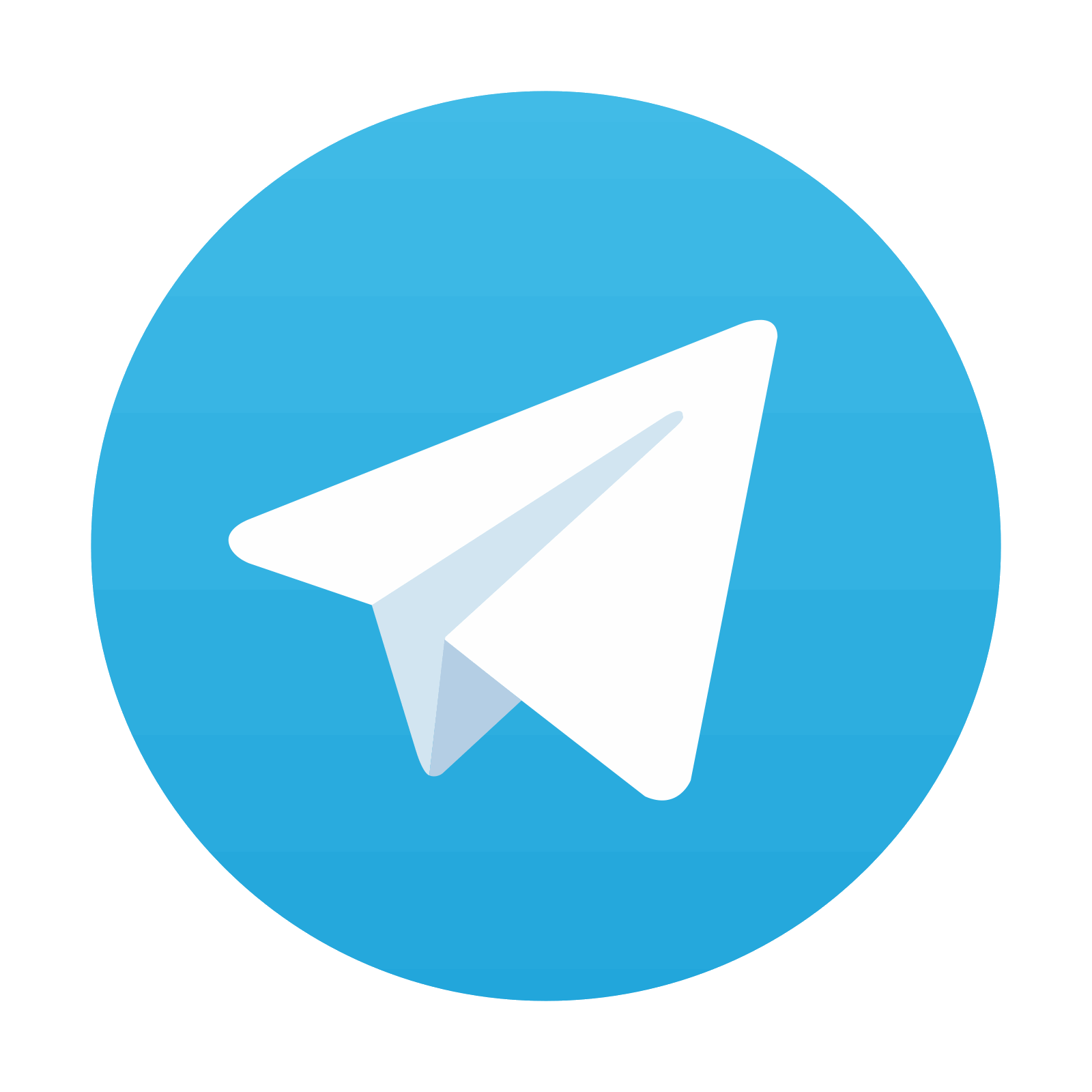
Stay updated, free articles. Join our Telegram channel
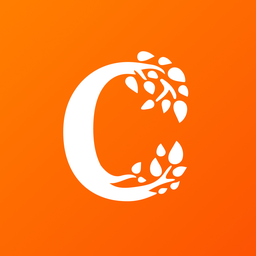
Full access? Get Clinical Tree
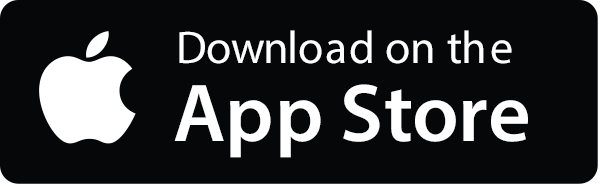
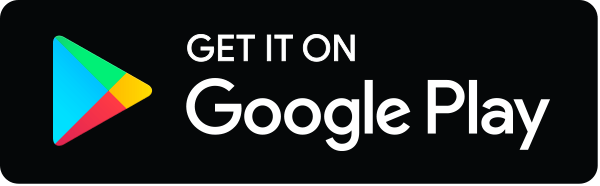