Pathophysiology and Management of Respiratory and Mixed Acid–Base Disorders
Respiratory acid–base disorders are caused by primary changes from normal excretion of carbon dioxide (CO2) by the lungs. Primary means that the changes are not secondary to changes in pH caused by metabolic acid–base disorders. Under usual metabolic conditions, the body makes 13,000 to 15,000 mmol of CO2 per day from the catabolism of carbohydrate, protein, and fat. If the lungs excrete this amount, the quantity of CO2 in the body remains the same. This is reflected by the amount of CO2 dissolved in blood and the partial pressure of the CO2 gas in equilibrium with it (PCO2). A small amount of the dissolved CO2 reacts with water to form carbonic acid (H2CO3), the acid part of the Henderson–Hassel balch acid–base equation discussed in Chapter 3 and in detail elsewhere (1). This relationship between PCO2 and pH is shown below:
If the lungs’ excretion does not match the daily production of CO2, the quantity of CO2 in the body changes; therefore, the amount dissolved in the blood and the pressure it generates (PCO2) change in the same direction. This change generates either of the two simple (or primary) respiratory acid–base disorders: hypercapnia, or high CO2 level, generates respiratory acidosis; hypocapnia, or low CO2 level, generates respiratory alkalosis.
The whole body responds to changes in CO2 content in a programmed fashion. In step 1, the pH change causes rapid chemical buffering. Buffers within cells either take up hydrogen ions [H+], producing a bicarbonate [HCO3−] in the blood, or give up H+ to titrate (consume) HCO3− in the blood. In step 2, the abnormal blood PCO2 alters the renal tubular cell PCO2, causing changes in H+ secretion that result in changes in renal net acid excretion (NAE) that, in turn, raise or lower blood [HCO3−]. This process is called by its traditional name of compensation, but it is more of an adaptation to a new state of CO2 balance. This process takes days to reach a new steady state. Thus, it occurs only in chronic respiratory acid–base disorders. In step 3, the respiratory system corrects the problem and restores the whole-body CO2 content and the arterial PCO2 (PaCO2) to previously normal values. Obviously, this can occur only if the causative disorder is cured or corrected. Notably, changes in oxygen (O2) supply and demand and the PaO2 do not define respiratory acid–base disorders, but they can cause both respiratory acid–base disorders and metabolic acidosis through their effects on respiratory drive and lactic acid metabolism (2).
Carefully obtained arterial blood for analysis is the usual way of diagnosing respiratory acid–base disorders. There are a variety of approaches used to correctly classify acid–base disorders (3). A commonly used approach is a physiologic one based on the Henderson–Hasselbach equation. To accurately identify an acid–base disorder using this approach, one needs measurements of pH, PCO2, HCO3, and the anion gap. This approach uses only the carbonic acid/bicarbonate buffer system to assess acid–base disorders and is the approach that will be used in this chapter. The two other approaches to acid–base disorders are the base excess approach and the physicochemical approach (Stewart approach). The former analysis takes into consideration all buffer systems in the body, whereas the latter approach measures the variables of strong ion difference and weak acid concentration. These two approaches have been reviewed extensively (3) and will not be discussed further in this chapter.
Respiratory Acidosis
Respiratory acidosis is a disorder caused by processes that increase PCO2, which thereby leads to a decrease in the pH. The PCO2 increases when the lungs fail to excrete metabolically produced CO2. A decrease in effective alveolar ventilation is the usual way that the PCO2 is increased. Effective alveolar ventilation can be diminished in two major ways, namely, decreased minute ventilation or ventilation–perfusion inequality (4). If effective ventilation is fixed by a ventilator or respiratory disease, the introduction of nutrition, parenteral or enteral, will increase the generation of CO2. Using glucose as the source of nonprotein calories increases CO2 production by 20% compared with glucose–lipid mixtures (5).
With decreased effective alveolar ventilation, CO2 excretion falls short of production, and the quantity of CO2 carried per milliliter of blood increases, as reflected in an increased PaCO2. When a steady state of hypercapnia is reached, the ventilatory excretion of CO2 again equals production. This new state occurs because a higher concentration of CO2 is carried to the pulmonary vascular bed, allowing more CO2 excretion.
When the PaCO2 rises, the amount of dissolved CO2 increases and shifts the equilibrium reaction to favor the production of H2CO3; thus, CO2 + H2O → H2CO3. This increased acid results in a fall in pH or respiratory acidosis. This process can be visualized more simply as a rise in PaCO2, which reduces the ratio of the HCO3− concentration to the PaCO2, thereby causing a fall in pH:
The chemistry of these reactions is discussed in Chapter 3.
PATHOPHYSIOLOGY OF RESPIRATORY ACIDOSIS
Buffering
The immediate response to the low pH generated by the increased PaCO2 and H2CO3 is to buffer (or bind) hydrogen ions with nonbicarbonate buffers. Bicarbonate does not work as an effective buffer in this situation because it reacts with hydrogen ions to form H2CO3, which is the original culprit. In the extracellular fluid (ECF) space, proteins constitute the only buffer, whereas within the cells, hemoglobin, phosphate, proteins, and lactate are the major nonbicarbonate buffers; 97% of the buffering of H2CO3 derives from intracellular rather than extracellular fluid buffers (6).
Renal Compensation
The definitive compensation for respiratory acidosis resides solely in the kidneys. The kidneys respond to the increased systemic PCO2 by increasing the production and excretion of ammonium (NH4+). The renal tubular cells metabolize glutamine to produce two NH3 molecules and α-ketoglutarate (AKG). The AKG goes to the liver, where metabolism produces two HCO3−. The kidney excretes the NH3 as NH4+, which marks the addition of the new HCO3− to the body stores by the liver. If the kidneys fail to excrete the NH4+, it travels to the liver, and metabolism generates H+, which negates the addition of the HCO3− to the body. Thus, increased renal excretion of NH4+ is a crucial component of the generation of new HCO3−. The increased urinary NH4+ excretion is balanced by increased Cl− excretion, with a resultant fall in plasma (Cl−). When a steady state of hypercapnia is reached, chloride excretion returns to normal and equals intake. NH4+ excretion also returns to normal, even though H+ secretion remains increased. The persistently increased H+ secretion is needed to reclaim the increased filtered HCO3− load that results from the increase in plasma concentration (7,8). Experimental evidence has shown that both the proximal tubule and the distal tubule participate in adaptation to respiratory acidosis. In the proximal tubule, increases in PaCO2 cause corresponding activation of the luminal Na/H exchanger and basolateral Na/HCO3 exchanger, leading to net reabsorbtion of bicarbonate (9). There also appears to be increased activity of H+/ATPase in the distal tubule (10). The chemical buffering and renal compensation that occur with chronic respiratory acidosis are diagramed in Figure 4-1.
Figure 4–1 Pathophysiology of chronic respiratory acidosis. Chemical buffering and renal compensation combine to elevate the plasma [HCO3−]. The renal mechanisms involve an adaptive increase in ammonium and chloride excretion until the new steady state is reached.
Correction of Respiratory Acidosis
The third, or corrective, response to respiratory acidosis is the restoration of effective ventilation. Correction or amelioration of an acute neurologic process causing hypoventilation or a ventilatory or gas-exchange defect may be possible. Unfortunately, many processes that result in chronic hypercapnia are caused by irreversible parenchymal lung damage and thus can be corrected only partially at best.
In metabolic acidosis, the corrective agent, the kidney, is sometimes a cause of the disorder, as in uremic acidosis, but at other times is not, as in diabetic ketoacidosis. In respiratory acidosis, however, the respiratory system, which includes neural control—mechanical, circulatory, and membrane exchange components—always is involved as the cause of the disorder and is also the corrective agent.
ACUTE RESPIRATORY ACIDOSIS (ACUTE HYPERCAPNIA)
Acute respiratory acidosis results from acute alveolar hypoventilation with a primary elevation of the PaCO2, when only the buffering defense has had time to come into play. Although buffering occurs almost immediately, the renal response does not exert a noticeable influence for 12 to 24 hours (11). This is the period of time in which pure acute respiratory acidosis is observed. An appropriate defense of pH in acute respiratory acidosis is characterized by an elevation of the plasma [HCO3−] by about 1 mmol/L (>24) for each 10 mm Hg acute increment in PaCO2 (>40) (12):
Clinical Features and Systemic Effects of Acute Respiratory Acidosis
The acute onset of hypercapnia is invariably accompanied by hypoxemia, which usually dominates the clinical picture. Depending on the underlying disorder and the state of consciousness, the patient may present with the signs and symptoms of acute respiratory distress, including marked restlessness, tachypnea, and marked dyspnea. As the process worsens, stupor and eventually coma develop. CO2 has vasodilating properties, and thus hypercapnia is associated with increased cerebral blood flow (13,14). This increase in blood flow to the brain probably accounts for the headaches and occasional signs of increased intracranial pressure that can occur with both acute and chronic hypercapnia (15,16). Severe acute respiratory acidosis can cause refractory hypotension through two mechanisms (17). First, cardiac contractility is reduced and cardiac output falls. Second, peripheral arterial smooth muscle relaxes, causing vasodilation and decreased systemic vascular resistance. Modest acute increases in PaCO2 (13–19 mm Hg) actually increase cardiac output as well as pulmonary artery pressure (18).
In intensive care units, hypoventilation is frequently induced in patients with severe lung injury and acute respiratory distress syndrome (ARDS). This strategy was shown to reduce mortality and ventilator time in the ARDSNet trial (19). Although the difference in PaCO2 was not large (5 mm Hg), this trial did suggest that the benefits of low tidal volume ventilation outweigh the risks of mild acute hypercapnia. It should be noted that many patients in the trial were treated with bicarbonate therapy to minimize the fall in blood pH.
Laboratory Findings in Acute Respiratory Acidosis
With acute respiratory acidosis, the arterial blood reflects the pathophysiologic state with an elevated PCO2, a moderately elevated plasma [HCO3−] (<30 mmol/L), and a low pH. If the patient is breathing room air, then the PaO2 is decreased. The venous serum electrolytes reveal a modestly elevated total CO2 content, with usually normal plasma concentrations of sodium, potassium, and chloride.
Causes of Acute Respiratory Acidosis
Some of the causes of acute respiratory failure, which leads to acute CO2 retention, are listed in Table 4-1.
Treatment of Acute Respiratory Acidosis
The key to treatment of acute respiratory acidosis is the restoration of effective ventilation. Modest amounts of sodium bicarbonate (NaHCO3) may be given intravenously to mitigate severe acidosis; the latter is only a holding measure to prevent the serious cardiovascular effects of marked acidemia until definitive therapy is established (20). Because equilibration of HCO3− across the blood–brain barrier is markedly slower than that of CO2 with bicarbonate administration, a delay in the correction of the cerebral pH may occur, and cerebrospinal fluid pH falls initially (21).
Table 4–1 Causes of Acute Respiratory Acidosis
Neuromuscular Abnormalities |
Brainstem injury High cord injury Guillain–Barré syndrome Myasthenia gravis Botulism Narcotic, sedative, or tranquilizer overdose Status epilepticus Postoperative hyponatremia with herniation |
Airway Obstruction |
Foreign body Aspiration of vomitus Laryngeal edema Severe bronchospasm |
Thoracic–Pulmonary Disorders |
Flail chest Pneumothorax Severe pneumonia Smoke inhalation Severe pulmonary edema |
Vascular Disease |
Massive pulmonary embolism |
Respirator-Controlled Ventilation |
Low rate and/or tidal volume Large dead space Total parenteral nutrition (increased CO2 production) |
CHRONIC RESPIRATORY ACIDOSIS (CHRONIC HYPERCAPNIA)
Chronic respiratory acidosis is caused by chronic decreased effective alveolar ventilation with a primary elevation of the PaCO2. The duration of the elevation of PaCO2 must be sufficient to permit adaptation of the renal mechanisms to be maximized. In dogs, a new steady state of blood acid–base values occurs 5 days after the onset of hypercapnia (11). The exact time interval needed to establish “chronic” hypercapnia in humans has not been established. A quantitative relationship has been described between the steady-state PaCO2 and the H+ concentration in patients with chronic hypercapnia. This relationship is linear and is described by a slope of about 0.25 nmol of H+ per 1 mm Hg increase in PaCO2 (22,23). A clinical guide for bedside use expresses the relationship between PaCO2 and plasma [HCO3−] in chronic hypercapnia as follows: For each increment of 10 mm Hg in PaCO2, the plasma [HCO3−] rises by 4 mmol/L, with a range of 4 mmol/L in either direction. The following formula summarizes this rule of thumb:
Clinical Features and Systemic Effects of Chronic Respiratory Acidosis
Patients with chronic respiratory acidosis exhibit few, if any, signs or symptoms related directly to CO2 retention and acidosis. However, papilledema and other neurologic disturbances have been described in several patients (22,23). These findings are not caused by the hypercapnia per se nor by the pH changes that mediate cerebral vascular reactivity through intracellular calcium. Rather, secondary changes in catecholamines are the likely causes (13). The signs and symptoms of the chronic pulmonary disease, with or without cor pulmonale, usually predominate. Chronic respiratory acidosis causes decreased bone mineralization, although to a lesser degree than does metabolic acidosis (24–26). This effect does not appear to be mediated by altered function of bone osteoclasts or osteoblasts and is not accompanied by hypercalciuria (27,28).
Laboratory Findings in Chronic Respiratory Acidosis
Arterial blood examination reveals a low pH (not <7.25 even with severe chronic CO2 retention), an elevated PaCO2, and an elevated plasma [HCO3−]. Thus, a blood pH <7.25 is a marker of imposed acute hypercapnia or metabolic acidosis. Plasma sodium and potassium concentrations are usually normal. Total plasma CO2 content is elevated, and plasma chloride concentration is reciprocally decreased. The anion gap is usually normal (22). In the absence of diuretic use or vomiting, these serum electrolyte findings should lead the clinician to check arterial blood gas values. The urine pH is usually acid.
Causes of Chronic Respiratory Acidosis
Chronic respiratory acidosis is seen most commonly in patients with chronic obstructive pulmonary disease (COPD). However, any condition that can lead to chronic retention of CO2 (27) will cause the same acid–base disturbance. Examples of such conditions are given in Table 4-2.
The epidemic of obesity has led to an increased prevalence of the obesity hypoventilation syndrome (OHS). Patients with OHS exhibit hypercapnia while awake (PaCO2 > 45 mm Hg), obesity (BMI > 30 kg/m2), and absence of alternative causes of hypoventilation. Most patients with OHS have sleep-disordered breathing and obstructive sleep apnea. The hypoventilation may be secondary to altered respiratory muscle mechanics and decreased ventilator drive (29,30). Patients with OHS commonly develop hypoxemia, pulmonary hypertension, and signs of right-sided congestive heart failure. In patients with nocturnal hypercapnia due to sleep apnea but daytime normocapnia, metabolic alkalosis may actually develop. Nocturnal hypercapnia can lead to renal generation of bicarbonate. People on low-salt diets (low chloride) are unable to excrete this bicarbonate and thus develop posthypercapnic metabolic alkalosis.
Table 4–2 Causes of Chronic Respiratory Acidosis
Neuromuscular Abnormalities |
Chronic narcotic or sedative ingestion Primary hypoventilation Pickwickian syndrome Poliomyelitis Diaphragmatic paralysis Hypothyroidism Sleep apnea syndrome |
Thoracic–Pulmonary Disorders |
Chronic obstructive pulmonary disease Kyphoscoliosis End-stage interstitial pulmonary disease |
Treatment of Chronic Respiratory Disease
Chronic respiratory acidosis can be corrected effectively only by restoring or improving the ability of the respiratory system to excrete CO2. Often, this is impossible because of an irreversible pathologic condition. However, adequate airway drainage, relief of bronchospasm, and treatment of pulmonary infections and congestive heart failure may lead to significant improvement. Because the arterial pH remains >7.25 even with chronic PaCO2 elevations to 110 mm Hg (31), the acidosis per se is not dangerous, although the patient is at more risk for serious acidemia if metabolic acidosis occurs. Attention to the maintenance of adequate O2 tension (PO2) is the critical need.
People with end-stage renal disease (ESRD) who also have COPD or another cause of chronic hypercapnia are unable to generate the usual amounts of bicarbonate for compensation in chronic respiratory acidosis. Thus, they may benefit from dialysis using a higher bicarbonate dialysate.
ACUTE HYPERCAPNIA SUPERIMPOSED ON CHRONIC RESPIRATORY ACIDOSIS
When a patient in a steady state of chronic hypercapnia suffers a new insult to his or her ability to excrete CO2, the PaCO2 rises acutely to a new level. Thus, the plasma [HCO3−] and blood pH are lower than predicted for a given chronic level of PaCO2. However, the change in pH is not as great as would be expected for a similar acute increment in PaCO2 occurring in a previously normal person. That is, the pH is better protected against an acute rise in PaCO2 when there is a background of chronic respiratory acidosis than it is with acute respiratory acidosis alone (32,33). The mechanism for this is not entirely clear but has been attributed partially to the physicochemical effect of the preexisting higher [HCO3−], which would reduce the fall in pH compared with that seen for a similar increment in PaCO2 in a patient with a normal [HCO3−]. In addition, the kidney rapidly increases H+ excretion when an acute rise in PCO2 is superimposed on chronic respiratory acidosis. This is in contrast to acute respiratory acidosis alone, in which renal acid excretion makes little quantitative contribution to H+ balance. Treatment is directed toward correcting the acute disorder and providing supplemental O2.
Respiratory Alkalosis
Respiratory alkalosis is caused by a process that leads to a rise in pH owing to a primary decrease in the PaCO2. The PaCO2 can fall only if the excretion of CO2 by the lungs exceeds the production of CO2 by metabolic processes. Because the production of CO2 usually remains relatively constant, a negative CO2 balance results primarily from increased alveolar ventilation. Hyperventilation can result from two processes: (a) increased neurochemical stimulation of ventilation by central or peripheral neural mechanisms and (b) physically increased ventilation, either artificially with mechanical ventilators or voluntarily with increased conscious effort. Alveolar hyperventilation produces increased CO2 excretion, which reduces the PaCO2 and H2CO3. This fall in PaCO2 increases the ratio of the [HCO3−] to PaCO2, which results in a rise in the pH of the blood, that is, alkalemia.
The buffering response constitutes the acute phase of respiratory alkalosis, whereas the renal response to hypocapnia defines the chronic stage of respiratory alkalosis.
PATHOPHYSIOLOGY OF RESPIRATORY ALKALOSIS
Buffering
Buffering constitutes the first response in respiratory alkalosis. To return the pH toward normal in the face of the decreased H2CO3 or PaCO2, the plasma [HCO3−] must be decreased. Therefore, H+ is released from the body buffers, and the plasma [HCO3−] is reduced by the following net reaction:
Intracellular buffers supply about 99% of the H+, whereas plasma proteins contribute about 1% to the buffering effort (6). Cellular metabolism contributes by increasing the production of lactic acid and possibly of slight amounts of other organic acids. Lactate concentration increased by 0.5 mmol/L in a study in anesthetized patients; this represents about 10% of the total buffering effort. Buffering is completed within minutes, and the steady state persists for at least 2 hours (34). The alacrity of the response is critical because the PaCO2 can decrease abruptly, and without buffering, life-threatening alkalemia would occur. The quantitative change in plasma [HCO3−] is not great, however, and the pH therefore may rise markedly. The arterial [HCO3−] fell to as low as 18 mmol/L at PaCO2 levels of 15 to 20 mm Hg in anesthetized patients (35). A rule of thumb for acute respiratory alkalosis is that the [HCO3−] should decrease by 1 mmol/L for each 10 mm Hg decrement in PaCO2:
Renal Compensation
The second adaptive response in respiratory alkalosis is handled by a renal mechanism. The kidney attempts to lower the plasma [HCO3−] in either of two ways: by decreasing the reclamation of filtered HCO3−, thus leading to bicarbonaturia, or by reducing the generation of new HCO3− to replace that consumed in the daily buffering of the dietary metabolic acid load. In animals, the kidney appears to make the second choice because a decreased NH4+ excretion without an increased HCO3− excretion occurs during the phase of adaptation to chronic hypocapnia (35). This reduction in excretion of NH4+, a cation, is balanced electrochemically by increased sodium or potassium excretion (36). After a new steady state is reached, excretion of these electrolytes returns to normal. The process of renal adaptation appears to occur rapidly and is probably completed within 24 to 48 hours (36). In humans, the early stage of renal adaptation for prolonged hypocapnia is characterized by bicarbonaturia, natriuresis, and decreased NH4+ and titratable acid excretion (37,38). The stimulus for this renal response appears to be independent of systemic pH changes but is a direct effect of the PaCO2 level on renal reabsorption of an anion, either HCO3− or chloride (39,40). The chemical buffering and proposed renal response in respiratory alkalosis are diagramed in Figure 4-2.
The quantitative contribution of the kidney to pH defense is difficult to judge in humans. Subjects with hypocapnia caused by voluntary hyperventilation or altitude hypoxemia had decreases in plasma [HCO3−] of 2.1 to 4.9 mmol/L/10 mm Hg decrease in PaCO2 during 1 to 11 days of hypocapnia (37,38,41). Arterial pH remained frankly alkalemic. Climbers adapted to altitude over 60 days had PaCO2 levels of 13.3 and [HCO3−] of 10.8 mmol/L with resultant pH of 7.53 just below the Everest summit (42). However, some studies of lifelong high-altitude dwellers have shown decreased plasma [HCO3−] sufficient to produce pH values of 7.4 with PaCO2 levels as low as 28 to 30 mm Hg (43–46). For clinical purposes, a useful rule is to diagnose simple chronic respiratory alkalosis when plasma [HCO3−] is decreased 4 mmol/L <24 for each 10 mm Hg chronic decrement <40 in the PaCO2.
Figure 4–2 Pathophysiology of chronic respiratory alkalosis. Intracellular buffers donate H+ to the ECF to produce a small decrease in plasma [HCO3−] during acute hypocapnia. The net effect on renal function of prolonged hypocapnia is a decrease in H+ secretion, which results in a fall in net acid excretion below the level necessary to maintain acid balance. Thus, plasma [HCO3−] level falls. Urinary acid and electrolyte excretion return to normal after steady-state PaCO2 and [HCO3−] are achieved. ECF, extracellular fluid; TA, titratable acid.
Correction of Respiratory Alkalosis
The third, or corrective, response in respiratory alkalosis entails correction of the hyperventilation that maintains the negative CO2 balance. This, of course, is dependent on removal of the neurohumoral stimulus to the respiratory center or cessation of mechanical or voluntary hyperventilation. The latter is easier to achieve because neural stimulation of ventilation is often caused by pathophysiologic processes that are difficult to correct.
CLINICAL FEATURES AND SYSTEMIC EFFECTS OF RESPIRATORY ALKALOSIS
Hypocapnia may be manifested by symptoms and signs of neuromuscular irritability. Patients may complain of perioral and extremity paresthesias, muscle cramps, and even tinnitus. Hyperreflexia, tetany, and even seizures may occur (47,48). Hypocapnia causes cerebral vasoconstriction with reduced blood flow, which may have deleterious and even fatal effects on the brain, especially in patients with sickle cell disease (49–52). Marked alkalemia may cause serious refractory cardiac arrhythmias and electrocardiographic changes of ischemia (53–57).
LABORATORY FINDINGS WITH RESPIRATORY ALKALOSIS
If arterial blood pH is increased, then a decreased PaCO2 and plasma [HCO3−] are diagnostic of respiratory alkalosis. Venous serum total CO2 content reflects the decrease in [HCO3−], and chloride concentration is slightly increased. Serum potassium is increased by an average of 0.3 mmol/L in acute respiratory alkalosis. This effect appears to be stimulated by the buffering-induced fall in [HCO3−], which activates the α-adrenergic system (Chapter 5) (58). Serum phosphorus concentration may decrease only slightly or to seriously low levels (Chapter 6) (59–61). Urine pH is not clinically informative. It may be relatively alkaline (>6.0) during the onset of acute hypocapnia but then fluctuates into the more acidic range, as in the eucapnic state (37).
DIFFERENTIAL DIAGNOSIS OF RESPIRATORY ALKALOSIS
Respiratory alkalosis is the most common acid–base disorder among seriously ill patients (62). The reason for this is apparent from a review of the list of causes of respiratory alkalosis (Table 4-3).
Central stimulation of the medullary respiratory center occurs with anxiety, pain, pregnancy, febrile states, and salicylate intoxication. Mechanical irritation by brain trauma or tumor is another respiratory stimulant.
Stimulation of the peripheral pathways to the medullary respiratory center occurs in pulmonary–thoracic disorders that cause hypoxemia with relatively unimpaired CO2 transport, altitude hypoxemia, asthma (63), and disorders that decrease lung compliance (stiff lung) without necessarily causing hypoxemia.
Mechanical ventilation can be associated with respiratory alkalosis if the rate and/or tidal volume are inappropriately high. While most forms of respiratory alkalosis are associated with mild alkalemia, this situation can often be associated with severe alkalemia (pH > 7.55) (64).
Patients with hepatic cirrhosis often have respiratory alkalosis (65). The mechanism is probably multifactorial (66), although increased pulmonary shunting (67), hyponatremia (68), and increased blood ammonia levels (69) have been implicated. Respiratory alkalosis is an early manifestation of gram-negative sepsis and other forms of shock; therefore, the clinician should suspect these processes in the appropriate clinical setting (70).
TREATMENT OF RESPIRATORY ALKALOSIS
The only definitive treatment is to correct or ameliorate the basic disorder responsible for the hyperventilation. Correcting significant hypoxemia is more critical for the patient’s well-being than is correcting the acid–base disturbance. If alkalemia is causing deleterious neuromuscular or cardiac rhythm problems in a patient on mechanical ventilation, then decreasing the minute ventilation or increasing the dead space may be effective. If this cannot be done without compromising oxygenation, then the use of an inhaled gas mixture containing 3% CO2 may be helpful for short periods of time (71).
Table 4–3 Causes of Respiratory Alkalosis
Central Stimulation of Respiration |
Anxiety Head trauma Brain tumors or vascular accidents Salicylates Fever Pain Pregnancy |
Peripheral Stimulation of Respiration |
Pulmonary emboli Congestive heart failure Interstitial lung diseases Pneumonia “Stiff lungs” without hypoxemia Altitude Asthma |
Multiple Mechanisms |
Hepatic insufficiency Gram-negative septicemia |
Mechanical or Voluntary Hyperventilation |
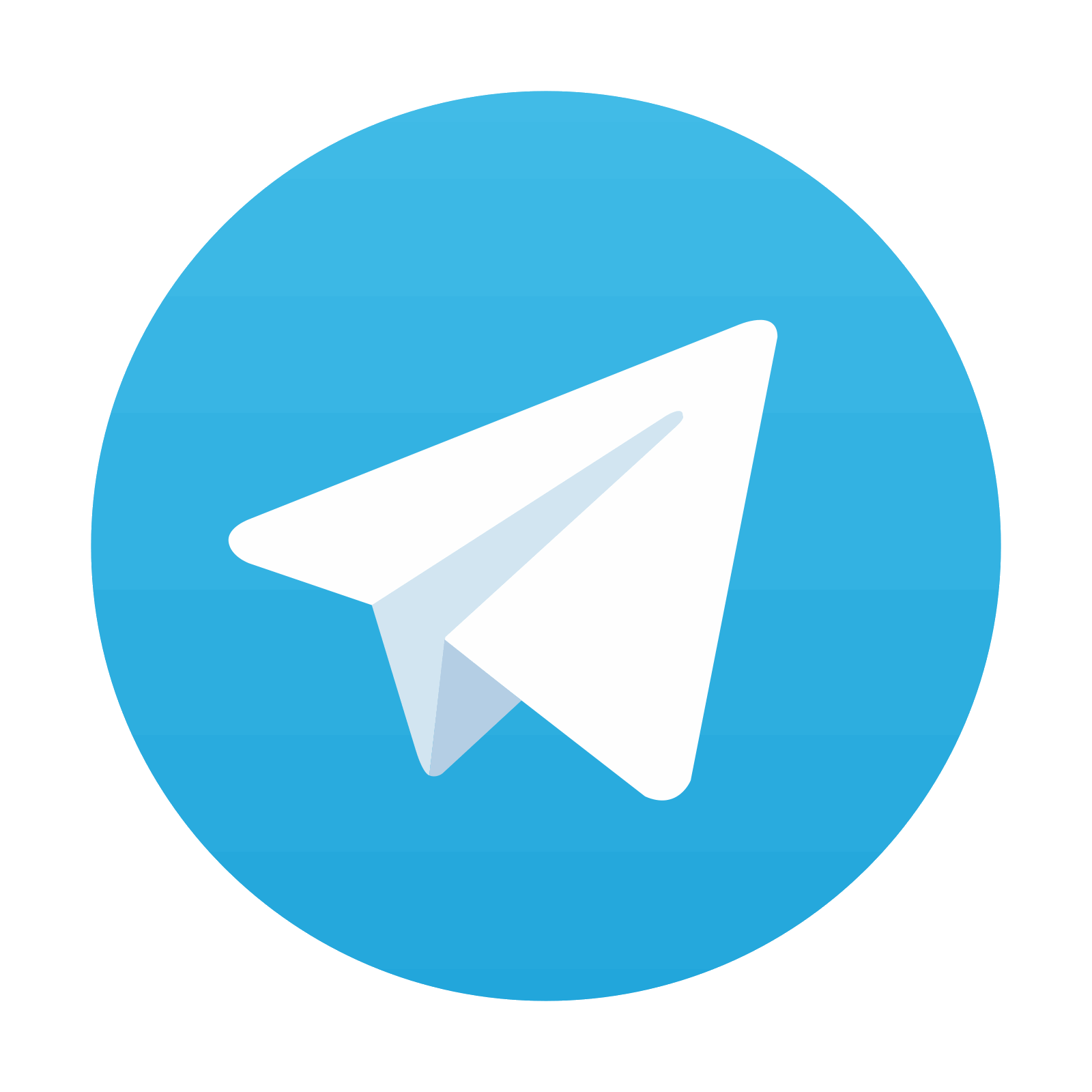
Stay updated, free articles. Join our Telegram channel
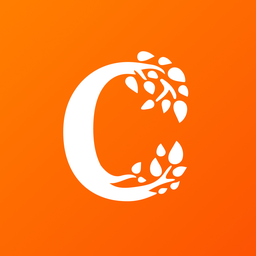
Full access? Get Clinical Tree
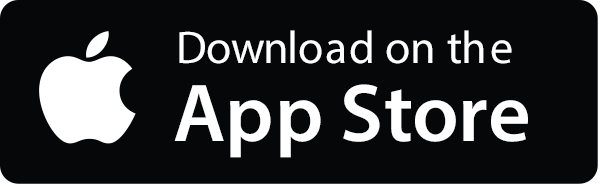
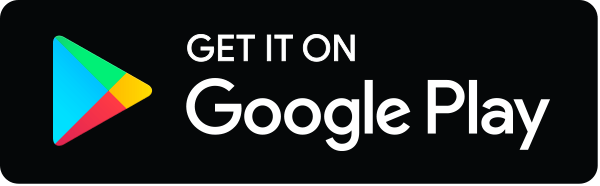