div class=”ChapterContextInformation”>
4. The Pathogenesis of Physical Frailty and Sarcopenia
Keywords
SarcopeniaCirrhosisProtein homeostasisAutophagyMitochondriaHyperammonemic stress responseIntroduction
Sarcopenia or skeletal muscle loss is one of the most frequent complications of cirrhosis that adversely affects survival and development of and response to other complications of cirrhosis, quality of life, and post-liver transplantation outcomes [1–4]. Increasingly, frailty, a compendium of clinical manifestations due to impaired contractile function, is associated with sarcopenia [4–6]. Despite the high clinical significance, there are limited therapeutic options and no consistently effective therapies to prevent or reverse sarcopenia because the mechanisms are poorly understood [2, 7, 8]. Nutritional interventions including supplementation have generally been ineffective because it is increasingly recognized that cirrhosis is a state of anabolic resistance, due to impaired response to physiological anabolic stimuli [9–12]. Mechanistic understanding of the causes of anabolic stimuli that will permit development of therapies targeting sarcopenia and contractile dysfunction requires direct studies on skeletal muscle from human and experimental models of liver disease. Skeletal muscle is the largest store of proteins in mammals and consists of both structural proteins that maintain muscle mass and contractile proteins that are responsible for locomotor function [13, 14]. Muscle mass is maintained principally by a balance between protein synthesis and protein breakdown or protein homeostasis (proteostasis) [14]. Since the skeletal muscle cells are terminally differentiated, in addition to protein homeostasis, satellite cells that are myogenically committed stem cells provide myonuclei for maintenance of muscle mass and nuclear accretion during recovery [15]. Even though there are extensive data on satellite cell biology, there is very limited data on satellite cell dysfunction in sarcopenia of disease, and loss of satellite cells does not consistently result in muscle loss [15–17]. Therefore, it is believed that dysregulated proteostasis is a major cause of sarcopenia and impaired contractile function in liver disease [2]. The underlying mechanisms of dysregulated protein homeostasis include defects in availability of substrates for protein synthesis, primarily amino acids and adenosine triphosphate (ATP), the principal cellular energy carrier generated in mitochondria, molecular signaling components that regulate protein synthesis and protein breakdown, and ribosomal components and function where protein synthesis occurs [8, 14]. Mediators of the nutritional, molecular, biochemical, and hormonal abnormalities that result in dysregulated protein homeostasis and organelle dysfunction in the skeletal muscle are being increasingly recognized and are potential therapeutic targets to reverse or prevent sarcopenia in patients with liver disease.
The main focus of this review will be on the pathogenesis of dysregulated skeletal muscle protein homeostasis and their potential mediators in cirrhosis that result in sarcopenia.
Impact of Terminology and Definitions in Identifying Pathophysiology of Sarcopenia
The term malnutrition in cirrhosis has been used in the past to refer to a constellation of nutritional deficiencies in patients with liver disease that adversely affected clinical outcomes [1, 8, 18]. However, a careful evaluation of these studies has shown that the principal component of malnutrition that affects clinical outcomes is the phenotype of skeletal muscle loss with or without fat loss and bioenergetic dysfunction [1, 19–23]. In contrast to the past when the phenotype of malnutrition consisted primarily of muscle loss with varying degrees of fat loss, the epidemic of obesity has made it difficult to clinically recognize malnutrition or sarcopenia in these patients [24–26]. Therefore, with the increasing availability of imaging techniques, muscle mass can be quantified with increasing precision and reiterates the previous studies that muscle loss is a critical predictor of clinical outcomes in patients with liver disease [8]. Multiple groups have reported criteria to define sarcopenia based on local population-based normative values [27–30]. This is critical because physiological studies have shown that major predictors of skeletal muscle mass depend on age, gender, and ethnicity, and normative values need to be defined based on these characteristics [26, 31, 32]. Since muscle loss occurs physiologically with aging after about 50 years of age, females have lower muscle mass, and age- and gender-specific values should be used to define sarcopenia [28, 31]. This is critical because identifying the mechanisms of sarcopenia and developing therapies and measures of response to interventions require appropriate measures of muscle mass. Currently, CT-based measures of the skeletal muscle index at the level of the 3rd lumbar vertebra are used to define sarcopenia in liver disease at most centers using published cutoff values [8, 29]. Even though loss of muscle mass is generally accompanied by impaired muscle contractile function, the term sarcopenia specifically refers to loss of muscle mass, while the term contractile dysfunction and frailty are used to define the disordered functional consequences in these patients [33, 34]. There is increasing recognition of the interplay between loss of muscle mass and impaired contractile function [35], and there may be shared and independent underlying mechanisms that cause sarcopenia and frailty. Unlike sarcopenia that has a defined phenotype, frailty is a constellation of functional abnormalities making it difficult to identify the pathogenesis [6, 34, 36, 37]. Therefore, the mechanisms of sarcopenia and contractile dysfunction are most likely to provide insights into the underlying pathogenesis of frailty.
Models of Sarcopenia in Liver Disease and Relevance to Mechanistic Studies
Common models used to study the pathogenesis of sarcopenia in liver disease
Model | Advantage | Limitations |
---|---|---|
Human cirrhosis | Most relevant to disease diagnosis and therapy | Variability between subjects Differences in etiology of liver disease Duration of disease is not known Hepatocellular dysfunction and vascular consequences contribute to sarcopenia Mechanistic studies are difficult to perform |
Portocaval anastomosis (only rat) | Hyperammonemia and low testosterone mimic perturbations in human cirrhosis Vascular consequences due to portocaval shunting on sarcopenia can be studied independent of the hepatocellular necroinflammatory responses of cirrhosis Duration of disease is known – time of surgery | No underlying cirrhosis Potential variability of shunt diameter No portal hypertension – the underlying causal factor for portocaval anastomosis |
Bile duct ligation (rat or mouse) | Liver is cirrhotic Hyperammonemia Increased circulating bile salts Genetic manipulations allow molecular dissection | Model of secondary biliary cirrhosis (a rare human disease) Confounding effects of bile salts and hyperammonemia on muscle protein turnover and signaling perturbations Steatorrhea and fat malabsorption cause severe calorie deficiency that affects muscle mass Onset of disease not certain – cirrhosis develops at varying times |
Toxin-induced liver fibrosis Carbon tetrachloride (phenobarbitone) Thioacetamide Dimethyl/ diethyl nitrosamine (rat or mouse) | Hepatic fibrosis Hyperammonemia Genetic manipulations allow molecular dissection | Variability in duration and severity of fibrosis with inconsistent cirrhosis Reversible fibrosis upon stopping toxin Direct toxin-induced muscle injury Hepatocellular carcinoma develops Inconsistent vascular consequences – portal hypertension and portocaval shunting |
Alcohol feeding models Acute Acute on chronic Chronic (rat or mouse) | Alcoholic liver disease Endotoxemia Fibrosis can occur Genetic manipulations allow molecular dissection | Variable response to ethanol Natural aversion of rodents to ethanol Ethanol effects on gut and liver confound muscle responses Direct effects of ethanol on muscle signaling and protein kinetics |
Myotubes (mouse/rat) | Specific mediators can be studied Subcellular organelle responses can be studied Genetic manipulations allow molecular dissection | In vitro system, cannot reproduce whole-body responses Physiological relevance needs to be established in in vivo studies |
Skeletal Muscle Proteostasis in Cirrhosis
Since skeletal muscle mass is regulated by a dynamic balance between protein synthesis and proteolysis, both metabolic and molecular alterations contribute to protein loss and ultimately sarcopenia. The metabolic regulation of protein synthesis and proteolysis depends on the underlying molecular regulatory pathways, and there is now increasing interest in identifying how metabolic perturbations are sensed by and alter the cellular molecular signaling pathways.
Whole-Body and Muscle Metabolism in Physiology and Disease

Postprandial and postabsorptive protein homeostasis. Prior to a meal (fasted/postabsorptive phase), protein synthesis is decreased and protein breakdown is increased and following a meal (postprandial state) these changes are reversed. Since postprandial protein synthesis restores proteolysis-mediated loss of protein content, protein homeostasis is maintained (blue hatched line), while in cirrhosis, the duration of postabsorptive phase is extended due to accelerated starvation with dysregulated proteostasis and progressive loss of protein content and muscle mass
Unlike the physiological postprandial (fed) state that lasts about 4–6 h after each meal, cirrhosis is a state of accelerated starvation [8, 50, 59]. Indirect calorimetry studies demonstrate a more rapid switch from a carbohydrate/glucose preference for energy (high respiratory quotient) to fatty acids as the major energy substrate with amino acid catabolism serving as gluconeogenic substrates (low respiratory quotient) [50, 57, 60]. In addition to the accelerated starvation physiology with proteolysis that occurs much earlier in cirrhosis after a meal than in controls, a significant proportion of patients with cirrhosis are hypermetabolic [61–63]. The underlying pathophysiology of hypermetabolism in cirrhosis is not clear but is associated with worse clinical outcomes [64, 65]. These data suggest that the duration of fasting, when proteolysis and muscle loss occur, lasts longer and the postprandial protein synthesis period is shorter in cirrhosis. In addition to the shorter duration of the postprandial phase and longer postabsorptive or fasting phase, cirrhosis is believed to be a state of anabolic resistance characterized by impaired responses to anabolic stimuli [7, 66, 67]. Therefore, the restoration of the muscle protein loss during the postprandial or fed state may not completely reverse the losses of the postabsorptive or fasting state resulting in a gradual but progressive muscle loss. Targeting the accelerated fasting in cirrhosis by frequent feeding and shortening the duration of the postabsorptive phase is a potential mechanistic therapeutic option to reverse and prevent worsening sarcopenia. The underlying mechanisms of these are currently under investigation, but cytokine and hormonal perturbations, including insulin resistance and endotoxemia, may contribute to these alterations.
Human Protein Kinetic Studies
A number of human studies have evaluated protein turnover with conflicting results. Increased, unaltered, and decreased rates of whole-body proteolysis and impaired protein synthesis have been reported using tracer kinetics [68–77]. This may be related to differences in etiology and severity of liver disease and duration of illness. A major limitation of these studies has been the lack of direct studies on the muscle since whole-body kinetics are influenced by organs besides the skeletal muscle. More recently, muscle protein synthesis measured using primed constant infusion and serial muscle biopsies before and following a single dose of branched chain amino acid supplementation showed similar rates of protein synthesis in cirrhosis and controls [78]. However, protein synthesis in the fasting state is currently not known.
Direct measures of muscle protein breakdown using tracer kinetics are challenging. 3-methyl histidine is formed by methylation of peptide-bound histidine in actin and myosin. After myofibrillar catabolism, 3-methyl histidine cannot be reutilized and is excreted in the urine. Studies using arteriovenous differences across the limb or urinary excretion of 3-methyl histidine used as measures of muscle protein breakdown also report conflicting results with increased, unaltered, or decreased muscle protein breakdown in cirrhosis [77, 79, 80].
Animal Studies
Decreased muscle mass with impaired muscle protein synthesis has been reported in the portocaval anastomosis rat and the bile duct-ligated rat [38, 81]. Direct measures of muscle protein breakdown in vivo are challenging. Instead, circulating amino acids and rate of appearance of specific metabolites have been used. There are no published data on direct measures of muscle protein breakdown using tracer kinetics in animals. Molecular measures of proteolysis have been used including altered ubiquitin proteasome pathway and autophagy [40, 41].
Cellular In Vitro Models
The best studied cellular system for a sarcopenic phenotype is the quantification of myotube size (diameter) in cellular cultures in response to potential mediators of muscle loss in cirrhosis [35, 40, 41, 46, 82–84]. These have been used to dissect the molecular and metabolic perturbations and have shown a reduction in protein synthesis, an increase in autophagy markers, and unaltered or decreased ubiquitin proteasome-mediated protein breakdown.
Molecular Perturbations in the Skeletal Muscle in Cirrhosis
In addition to direct studies in the skeletal muscle signaling pathways that regulate protein homeostasis, there is increasing interest in the regulation of these signaling perturbations in sarcopenia of liver disease [14, 59, 85]. Protein synthesis is regulated by two principal regulatory factors: myostatin, a transforming growth factor β superfamily member that inhibits protein synthesis, and locally generated insulin-like growth factor 1 (also known as mechanogrowth factor) that stimulates protein synthesis [2, 8, 40]. Canonical regulation of protein synthesis occurs via the Akt/mTORC1 that increases and the eukaryotic initiation factor 2α dependent regulatory pathway that inhibits protein synthesis [86]. A number of proteolytic pathways have been identified in the skeletal muscle and include the ubiquitin proteasome pathway, the lysosomal autophagy pathway, and the calcium-dependent calpain pathway of which there is robust literature in the former two proteolytic pathways in the muscle from models of liver disease [41, 45]. Perturbations in a number of upstream mediators including hyperammonemia, endotoxin, hormones, and nutrients cause dysregulation of these signaling pathways with impaired proteostasis and consequent sarcopenia [8, 14, 87, 88]. Understanding these perturbations is gaining increasing clinical interest because of the increasing availability of therapeutic options to restore proteostasis as a targeted approach to treat sarcopenia in liver disease.
Mediators of the Liver-Muscle Axis
Cirrhosis and other forms of liver disease with loss of muscle mass result in a number of biochemical, hormonal, cytokine, and metabolic perturbations that alter molecular signaling responses in the muscle culminating in dysregulated proteostasis and loss of muscle mass [7, 8]. Some of these have been studied directly in patients and models of cirrhosis, while others have been studied in non-hepatic disorders but are expected to result in activation of similar molecular perturbations. Of these, hyperammonemia is one of the best characterized mediators of the liver-muscle axis [89].
Physiologically, ammonia is a cytotoxic molecule generated mainly by gut bacterial metabolism as well as endogenous amino acid catabolism and purine breakdown during exercise in the skeletal muscle [90, 91]. Hepatocytes are the primary mechanism of ammonia disposal by the formation of urea, an energy requiring reaction [92]. In cirrhosis, ureagenesis is decreased due to hepatocellular dysfunction and portosystemic shunting with resultant hyperammonemia [93–95]. Even though hepatic encephalopathy is the best recognized adverse consequence of hyperammonemia [85], more recently the role of skeletal muscle in ammonia disposal and its consequences have been identified [96]. In a healthy subject, muscle is a net exporter of ammonia during fasting and exercise due to amino acid and purine catabolism with little clinical consequences because of normally functioning hepatocytes. In cirrhosis, multiple groups have reported an increased muscle uptake of ammonia [97, 98]; muscle ammonia uptake was generally believed to be a benign process. However, in the past decade, it is increasingly recognized that the skeletal muscle accumulates ammonia and functions as a metabolic partner to the liver [40, 41, 82]. Accumulation of ammonia in the skeletal muscle is not benign and initiates a series of metabolic, molecular, and organelle dysfunction with dysregulated protein homeostasis and potentially contributing to anabolic resistance [14, 40, 41]. Emerging data suggests that ammonia uptake by the transporter, RhBG, is increased by ammonia itself as well as causes of liver disease including ethanol [99].
Other potential mediators of the liver-muscle axis include endotoxemia with altered toll-like receptor signaling-mediated dysregulated protein homeostasis and muscle cell regeneration [87, 100], hormonal abnormalities including altered growth hormone secretion or resistance, and hypogonadism with decreased testosterone [101–103] and altered nutrient intake [104] and decreased physical activity [105]. This is however not a comprehensive list of potential mediators of the liver-muscle axis but includes those that have been evaluated in the development of sarcopenia. Other complications of cirrhosis including gastrointestinal bleeding, encephalopathy, ascites, renal dysfunction, and development of hepatocellular malignancy also aggravate the adverse consequences of the liver-muscle axis on skeletal muscle proteostasis, and their interaction with or contribution to the mediator (s) of the liver-muscle axis is not well characterized. Targeting the mediators of the liver-muscle axis is critical to reverse anabolic resistance, restore proteostasis, and improve muscle mass and function in cirrhosis.
Hormonal Perturbations in Cirrhosis
Male patients with cirrhosis and the PCA rat have lower circulating testosterone [106, 107]. Testosterone supplementation increases muscle mass in male patients with cirrhosis [103]. One of the potential mechanisms of testosterone is by a reduction in myostatin expression, but a more rapid aromatization of testosterone can accelerate its conversion to estradiol and impair beneficial responses [108]. Long-term safety of testosterone is also a concern, but given that testosterone or other aromatase resistant androgenic agents can inhibit muscle myostatin and protein synthesis, low androgens may be a potential mediator of decreased muscle protein synthesis in cirrhosis.
Growth hormone resistance or loss of the periodicity of growth hormone secretion has been reported in cirrhosis [102, 109, 110]. Growth hormone activates local insulin-like growth factor 1 and inhibits myostatin, and loss of growth hormone signaling may contribute to muscle loss [111].
Endotoxemia in Cirrhosis
Endotoxin is the lipopolysaccharide molecule in Gram-negative bacteria that is toxic to organisms primarily by the activation of host immune responses [112]. Low-grade sepsis and circulating endotoxemia have been reported in cirrhosis and patients with liver disease that can mediate muscle loss [87, 113, 114]. Endotoxin has been reported to impair protein synthesis by reduction in translational efficiency [12, 115]. The mechanistic basis of LPS-induced muscle loss is suggested to be mediated via a toll-like receptor (TLR)-4-dependent signaling with increased P65NFkB signaling, activation of inflammatory responses, and initiation of a cascade of molecular events that culminate in dysregulated proteostasis and muscle loss [100]. Even though overt sepsis may not be common, the low-grade endotoxemia due to a combination of increased gut permeability and impaired hepatic clearance of lipopolysaccharide has been referred to as metabolic endotoxemia [8]. Metabolic endotoxemia has been shown to impair muscle recovery responses and contribute to atrophy [116]. However, whether metabolic endotoxemia causes sarcopenia of cirrhosis and if the potential benefits of long-term antibiotics outweigh the risks are not known. Novel approaches to modulate TLR4 signaling and approaches to control metabolic endotoxemia are needed.

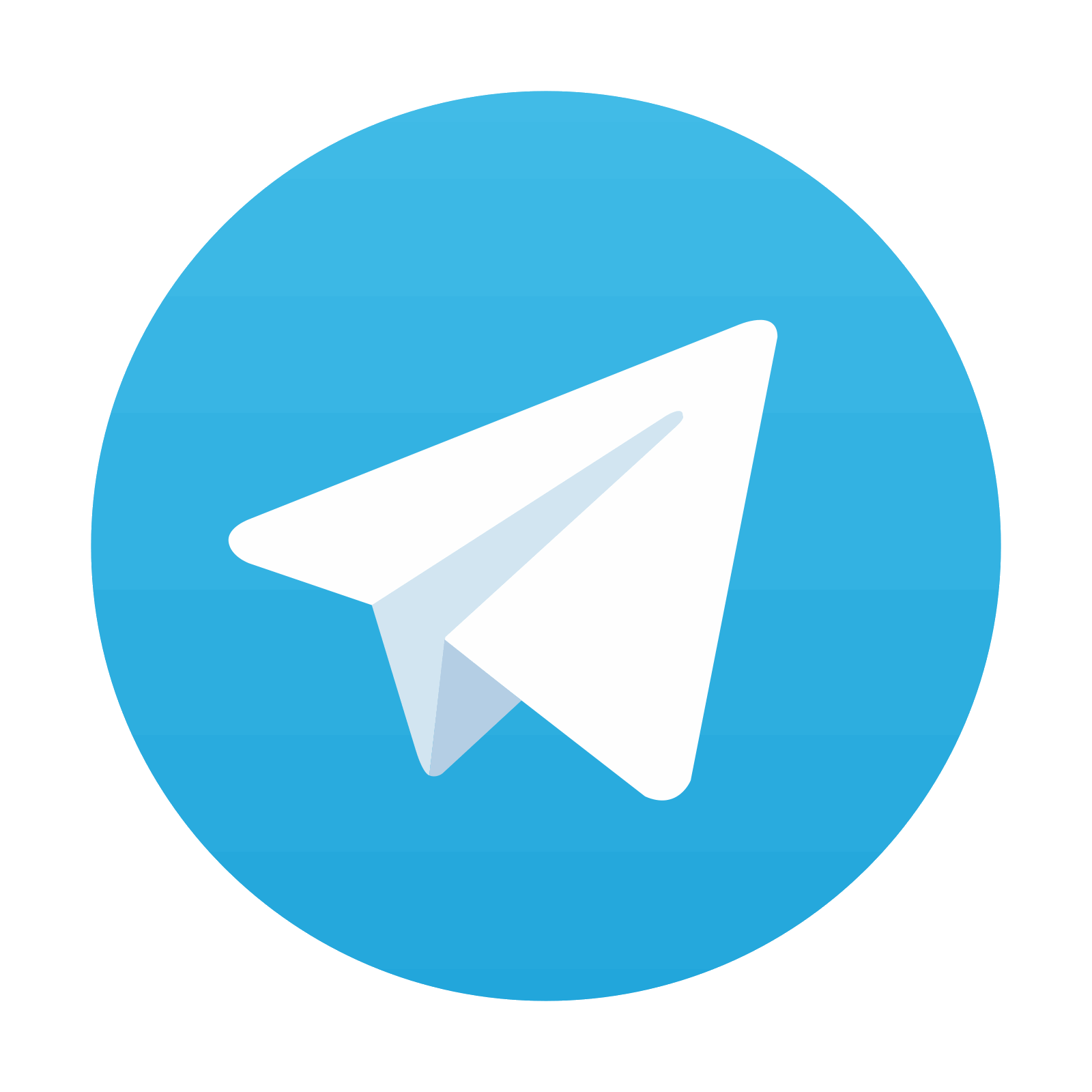
Stay updated, free articles. Join our Telegram channel
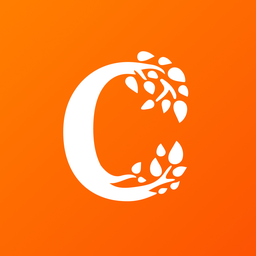
Full access? Get Clinical Tree
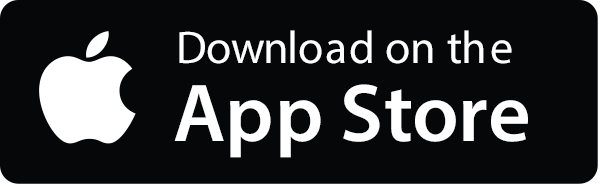
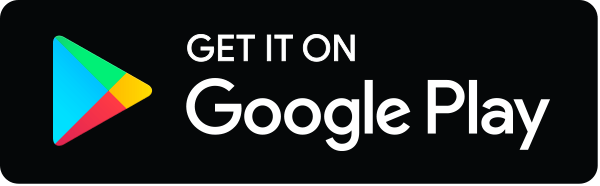