

important point, suppose 1 mL of 0.1 mol/L HCl is added to 9 mL of distilled water, the [H+] would increase from 10-7 to 10-2 mol/L. In other words, the pH would fall from 7.0 to 2.0. However, if we added 1 mL of 0.1 mol/L HCl to 9 mL of a 1 mol/L phosphate buffer (pK = 6.9) at pH 7.0, most of the dissociated H+ from HCl would combine with dibasic phosphate (HPO42-) and only slightly change the ratio of dibasic to monobasic (H2PO4–) phosphate. In fact, the pH would fall by only about 0.1 pH units. The addition of acid has been buffered by the phosphate dissolved in water. Another way to think about this is that the pH was stabilized by substances that bound the free H+ released by the HCl, in this case the phosphate. Such substances are called buffers (4).





in other words, to consider acids as electron acceptors rather than proton donors. In concrete terms, acid is generated when a substrate is metabolized to something more anionic (e.g., glucose is metabolized to lactate through the Embden-Meyerhof glycolytic pathway). Conversely, if a substrate is metabolized to something more cationic (e.g., lactate is metabolized to CO2 and H2O via the tricarboxylic acid [TCA] cycle), then acid is consumed (9). Because of the importance of the bicarbonate buffer system in the overall acid-base homeostasis, we generally consider the addition of a proton as equivalent to the decrease in total body HCO3– and loss of a proton as a gain in HCO3– (9).
![]() Figure 3-1 Modified Henderson-Hasselbalch equation, portraying the interacting effects of the primary acid-base disturbance and the secondary mechanisms on the pH. |
acid load must be excreted by the kidney to maintain acid-base homeostasis. The easiest way to understand the molecular processes involved in renal acid excretion is to separate renal acid-base handling itself into two functions: bicarbonate reabsorption and net acid excretion (NAE) (12).
sodium-hydrogen exchanger isoform 4 (NHE4) to the tubular interstitial space (32). This ultimately results in the increase in the medullary concentration of NH4, which is highest in the inner medulla. This NH4+ is then taken up by distal convoluted tubule and collecting duct cells, substituting for K+ using the basolateral Na+/K+ ATPase of the inner medullary collecting duct or as NH3 (after losing H+) utilizing ammonia-specific transporters Rhesus Glycoproteins (Rhbg and Rhcg) along with a component of diffusive absorption. Collecting duct ammonia secretion involves parallel secretion of NH3 utilizing Rhcg of the apical membrane or by its diffusive capacity along with cytosolic H+ generated by carbonic anhydrase II-mediated mechanism. H+ secretion across the apical membrane is primarily mediated by H+-ATPase and H+-K+ ATPase pumps. In the tubular lumen, H+ titrates luminal NH3 to form NH4+ and maintains low NH3 concentration in the urinary space, which is necessary for continued NH3 secretion (33). The net generation of any HCO3– from αKG metabolism is ultimately dependent on the excretion of NH4+. This is because if this NH4+ molecule is not excreted in the urine but rather is returned via the systemic circulation to the liver, it is used to form urea at the expense of generating an H+. Thus, the HCO3– molecule that was generated by the metabolism of the αKG will be neutralized, and no change in acid-base status will occur (30).
acid-base disturbance. Conversely, an exaggerated fall in PaCO2 producing a normal pH indicates the presence of respiratory alkalosis in the setting of a complex or mixed acid-base disturbance (44). The mechanism by which metabolic acidosis induces hypocapnia appears to be mediated in part by peripheral pH receptors in the carotid body, but mostly by central nervous system (CNS) pH receptors. This point is supported by the time course, specifically the temporal delay observed for respiratory compensation seen in experimental metabolic acidosis (45). The degree of chronic compensation varies from person to person; however, based on a large volume of clinical data, we can state with some confidence that the appropriate fall in PaCO2 (in torr) should be 1 to 1.5 × the fall in [HCO3–] (in mM) (44). Oral acid loading in normal subjects produced a rapid fall in PCO2 that reached a steady state after 30 minutes that was 0.85 times the fall in HCO3–, thus providing direct evidence for the rapid respiratory response to metabolic acidosis in humans (46).
of increases in prostaglandin E2 (PGE2) production (64). This aspect is extremely important in determining treatment of renal tubular acidosis (RTA) or the acidosis of chronic renal failure.





Table 3-1 Differential Diagnosis of Metabolic Acidosis | ||||||||||||||||||||||||||||||||||
---|---|---|---|---|---|---|---|---|---|---|---|---|---|---|---|---|---|---|---|---|---|---|---|---|---|---|---|---|---|---|---|---|---|---|
|
while referring to this nomenclature. RTAs can be divided into those characterized by disturbed distal nephron function (i.e., impaired NAE) and those caused by impaired proximal HCO3– reabsorption (82). Distal RTAs are divided into those associated with hypokalemia (83) and those associated with hyperkalemia, which may be further subdivided into RTA caused by hypoaldosteronism and RTA characterized by a general distal tubular defect (84).
Table 3-2 Causes of Renal Tubular Acidosis | |||||||||||||||||||||||||||||||||||||||
---|---|---|---|---|---|---|---|---|---|---|---|---|---|---|---|---|---|---|---|---|---|---|---|---|---|---|---|---|---|---|---|---|---|---|---|---|---|---|---|
|
in the urinary collecting system (i.e., renal pelvis, ureters, and urinary bladder), where the surface area for CO2 absorption is small. This CO2 essentially is trapped, and, when normalized for the blood PCO2 (i.e., the difference between urine and blood), is a marker for the rate of distal H+ secretion. Patients with back leak or pump failure generally have a small difference between urine and blood PCO2 (<20 torr).
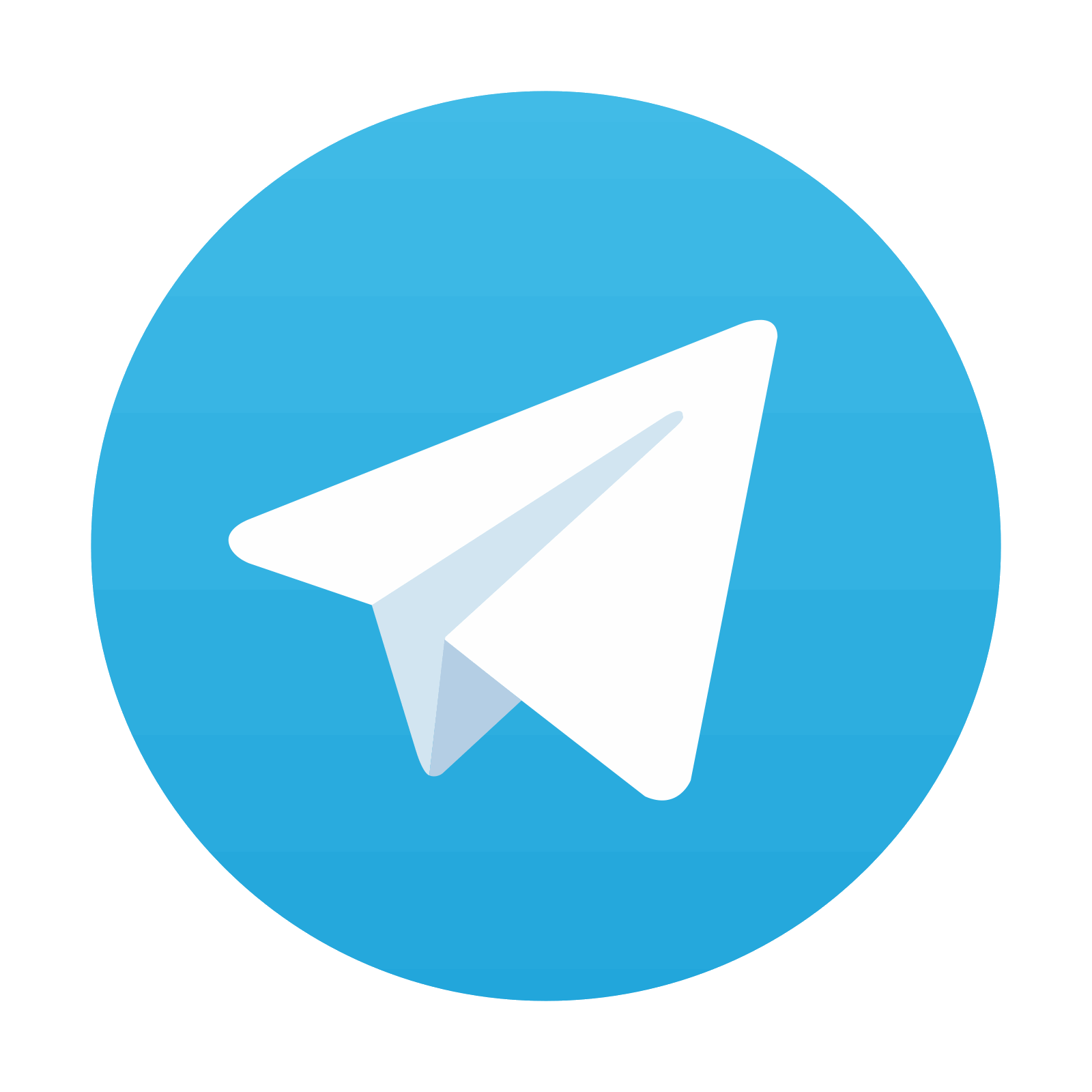
Stay updated, free articles. Join our Telegram channel
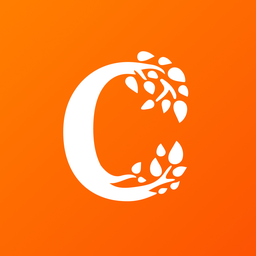
Full access? Get Clinical Tree
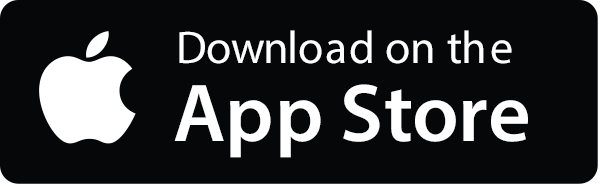
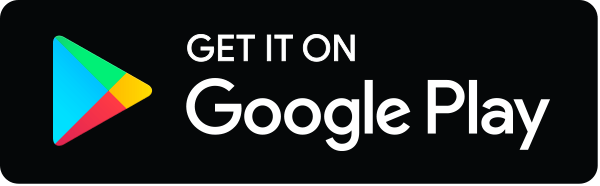
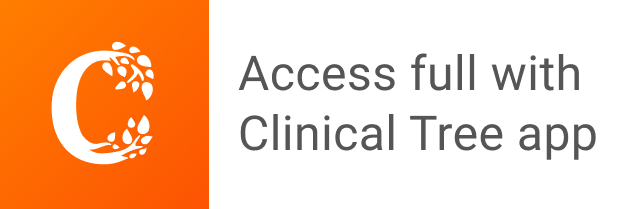