Fig. 4.1
Nuclear receptors regulate bile acid metabolism. Nuclear receptors that regulate expression of genes involved in bile acid metabolism are shown. FXR, PXR, and VDR respond to bile acids. SHP does not have a DNA-binding domain and represses expression of CYP7A1 and CYP8B1 as a corepressor. FXR, PXR, and VDR repress expression of CYP7A1, CYP8B1, or NTCP through indirect mechanisms
Nuclear receptors, including LXR, FXR, PXR, CAR, and VDR, have a structure comprised of an activation function 1 (AF1) region, a DNA-binding region with a C4-type zinc finger structure, a hinge region, and a ligand-binding domain containing an AF2 region [3]. Ligand binding alters the AF2 surface, leading to dissociation of a corepressor complex and recruitment of a coactivator complex [7]. These structural rearrangements allow the receptors to induce transcription of specific target genes. Nuclear receptors also exhibit transrepression effects and non-genomic actions through poorly characterized mechanisms. Both steroid hormones and bile acids are steroid compounds synthesized from cholesterol and act as ligands of nuclear receptors. Bile acids are essential factors for the ingestion and intestinal absorption of hydrophobic nutrients, such as cholesterol, fatty acids, and lipid-soluble vitamins, including vitamin D [8], and act as “prototypes” of steroid hormones by binding to nuclear receptors.
4.2 Bile Acid Metabolism
Primary bile acids, such as cholic acid (CA) and chenodeoxycholic acid (CDCA), are generated from cholesterol in the liver and are secreted in bile as glycine and taurine conjugates (in humans; glyco-CA, tauro-CA (TCA), glyco-CDCA, and tauro-CDCA (TCDCA)) [9, 10]. The synthesis of bile acids is mediated by at least 16 enzymes. The first step for cholesterol catabolism is initiated by one of two mechanisms, the classic pathway and the alternate pathway [9] (Fig. 4.2). In the classic pathway, cholesterol is converted to 7α-hydroxycholesterol by cholesterol 7α-hydroxylase (CYP7A1), a microsomal P450 enzyme. In humans, the classical pathway accounts for more than 50% of total bile acid production. In the alternate pathway, cholesterol is converted to 25-hydroxycholesterol and 27-hydroxycholesterol prior to being 7α-hydroxylated by oxysterol 7α-hydroxylase (CYP7B1). CYP27A1 hydroxylates cholesterol to 27-hydroxycholesterol and, to a lesser extent, 25-hydroxycholesterol, while a non-P450 enzyme, cholesterol 25-hydroxylase, produces 25-hydroxycholesterol. The alternate pathway is thought to produce less than 10% of the total bile acids under physiological conditions.


Fig. 4.2
Bile acid synthesis, enterohepatic circulation, and catabolism through the xenobiotic metabolic pathway. Nuclear receptor target gene products involved in bile acid metabolism are shown. Primary bile acids are synthesized by several enzymes, including CYP7A1 and CYP8B1, in the liver. Conjugated bile acids are secreted into bile via the canalicular transporter BSEP. Most bile acids are reabsorbed in the intestine, which expresses transporters, ASBT and OSTα–OSTβ, and an intracellular binding protein, I-BABP, and enter the enterohepatic circulation via portal circulation and hepatic uptake via NTCP and OATPs. Bile acids that escape reabsorption are converted to the secondary bile acids by intestinal bacteria. These bile acids are detoxified by CYP3A4 and SULT2A1. PAPSS2 provides the sulfate donor PAPS to SULT2A1. Hepatocytes can dispose bile acids through xenobiotic metabolism enzymes, such as CYP3A4 and SULT2A1, and basolateral transporters, MRP3, MRP4, and OSTα–OSTβ, leading to excretion into urine via renal transporters, MRP2, MRP4, and OSTα–OSTβ. FGF15/FGF19 is a hormone that regulates bile acid synthesis
The initial step of 7α-hydroxylation of sterol precursors is followed by modification of ring structures, side chain oxidation, and conjugation with taurine or glycine. The ring structure modification catalyzed by sterol 12α-hydroxylase (CYP8B1) finally produces CA, while the CYP8B1-independent pathway results in CDCA. CYP27A1, an enzyme that is involved in the alternate pathway, is also involved in the side chain oxidation of all intermediates regardless of their source. Oxidation by CYP27A1 is followed by side chain shortening and finally conjugation with taurine or glycine. In mice, CDCA is converted to α-muricholic acid (αMCA) and βMCA by unknown mechanisms.
Conjugated primary bile acids are secreted into the bile via the bile salt export pump (BSEP; ABCB11), an ATP-binding cassette (ABC) transporter that is localized in the canalicular membrane of hepatocytes [11] (Fig. 4.2). As the major components in bile, bile acids solubilize dietary lipids and promote their digestion and absorption in the small intestine. About 90–95% of conjugated bile acids are reabsorbed in the intestine and recirculate to the liver through the portal vein in a mechanism called the enterohepatic circulation. Less than 5% of bile acids escape reabsorption and are subjected to deconjugation, 7α-dehydroxylation, and other modifications by intestinal microflora, yielding the secondary bile acids, such as deoxycholic acid (DCA) and lithocholic acid (LCA) [12]. A portion of the secondary bile acids can enter the enterohepatic circulation from the ileum and colon and enter the bile acid pool. Because taurine conjugation is predominant in rodents, the major bile acids in mouse bile are TCA, tauro-αMCA (TαMCA), TβMCA, TDCA, and TωMCA [13, 14]. Ursodeoxycholic acid (UDCA) is generated from CDCA by intestinal bacteria [12, 15]. Interestingly, UDCA and tauro-UDCA (TUDCA) concentrations are higher in germ-free mice than in conventional mice, suggesting the presence of UDCA-generating enzyme(s) in mouse hepatocytes [14, 16].
Intestinal bile acid reabsorption is mainly mediated by the apical sodium-dependent bile salt transporter (ASBT; SLC10A2) expressed in the terminal ileum [11] (Fig. 4.2). Intracellular bile acids are bound to the intestinal bile acid-binding protein (I-BABP), then shuttled to the basolateral membrane, and effluxed into the portal circulation via the heterodimer organic solute transporter-α (OSTα) and OSTβ [4, 17]. The majority of circulating bile acids are taken up by hepatocytes via the Na+/taurocholate-cotransporting polypeptide (NTCP; SLC10A1) and organic anion-transporting polypeptides (OATPs). In addition to canalicular excretion by BSEP, the basolateral (sinusoidal) transporters multidrug resistance-associated protein 3 (MRP3), MRP4, and OSTα−OSTβ play a role in the alternative excretion of bile acids from hepatocytes into the systemic circulation. Renal MRP2, MRP4, and OSTα−OSTβ are thought to be involved in urinary bile acid excretion [18, 19].
4.3 LXR and FXR
Bile acids have been identified as regulatory signaling molecules for the transcription of genes involved in their synthesis (e.g., CYP7A1, CYP8B1) and transport (e.g., BSEP, NTCP). LXRα, originally identified as an orphan receptor, is activated by oxysterol intermediates in the bile acid synthetic pathway, such as 7α-hydroxycholesterol, 25-hydroxychoelsterol, and 27-hydroxycholesterol, while the more potent oxysterol for LXRα, 24(S),25-epoxycholsterol, is derived in a shunt pathway of cholesterol biosynthesis [20, 21]. Oxysterol-activated LXRα induces the transcription of mouse Cyp7a1, the rate-limiting enzyme in the classic pathway of bile acid synthesis by binding to a specific promoter element that consists of a two-hexanucleotide (AGGTCA or a related sequence) direct repeat motif separated by four nucleotides (direct repeat 4; DR4) [22] (Fig. 4.1). The ABC transporters ABCG5 and ABCG8, which mediate biliary excretion of cholesterol, are also induced by LXRα activation. A high cholesterol diet increases bile acid pool size in wild-type mice but not in LXRα-null mice [21]. Thus, LXRα plays a role in the feed-forward induction of bile acid synthesis in rodents. The expression of the human CYP7A1 gene is not regulated by LXRα due to an alternate sequence in the DR4 element [23].
LXRα acts as an RXR heterodimer, which can be activated by ligands for either LXRα or RXR [3]. According to this characteristic of the LXRα−RXR heterodimer, it had been predicted that treatment of mice with an RXR agonist could increase hepatic Cyp7a1 expression. Surprisingly, contrary to this prediction, RXR agonist treatment decreases Cyp7a1 expression in both wild-type and LXRα-null mice [24]. This finding led to the hypothesis that a distinct RXR heterodimeric partner might respond to bile acids, since bile acids have been known to induce feedback regulation in their synthesis by repressing Cyp7a1 expression [9]. Indeed, FXR was found to function as a bile acid receptor [25]. FXR was originally characterized as an orphan receptor that is weakly activated by farnesol, an intermediate in cholesterol synthesis, and juvenile hormone III, an insect hormone [26]. FXR belongs to the NR1H nuclear receptor subfamily along with LXRα; is expressed in the liver, intestine, kidney, and adrenal gland; and recognizes a two-hexanucleotide (AGGTCA or a related sequence) inverted repeat motif with one spacer nucleotide (inverted repeat 1; IR1) as an RXR heterodimer [5]. FXR is activated by both primary and secondary bile acids in their free and conjugated forms with relative potency CDCA > DCA = LCA > CA, but not by αMCA, βMCA, or UDCA [25, 27, 28] (Fig. 4.1). LCA, UDCA, TαMCA, and TβMCA have been reported to act as FXR antagonists [16, 29–31].
FXR regulates the synthesis and enterohepatic circulation of bile acids by both direct and indirect mechanisms [4, 32]. The orphan nuclear receptors HNF4α and LRH-1 are involved in transcription of the bile acid synthetic enzymes CYP7A1 and CYP8B1 [4, 33, 34] (Fig. 4.1). FXR represses the expression of CYP7A1 and CYP8B1 by inducing the transcriptional repressor SHP [35–38]. FXR appears to also repress expression of CYP7A1 and CYP8B1 via a SHP-independent mechanism, because significant Cyp7a1 and Cyp8b1 repression is retained in SHP-null mice fed cholic acid [38]. FXR induces the expression of fibroblast growth factor 19 (FGF19; or FGF15, the mouse ortholog of human FGF19) in enterocytes. FGF15/19 represses the expression of CYP7A1 by binding to a heterodimeric receptor composed of FGF receptor 4 and β-Klotho in hepatocytes through both SHP-dependent and -independent mechanisms [34, 39–41]. There are IR1 elements identified in human SHP and FGF19 genes [35, 42]. Thus, FXR activation suppresses bile acid synthesis through multiple mechanisms.
The hepatic bile acid transport system is also regulated by FXR. The basolateral transporter NTCP is negatively regulated by FXR through a SHP-mediated mechanism [37, 43], while its expression is induced by retinoic acid receptor and HNF4α [44, 45] (Fig. 4.1). The canalicular transporter BSEP is induced by FXR activation [37]. Human BSEP contains an IR1 element in the promoter [46]. FXR activation by a synthetic ligand protects hepatocytes from cholestatic liver damage by repressing bile acid synthesis and hepatocellular uptake and stimulating bile acid export from cells [47]. Despite this finding, FXR-null mice exhibit resistance to obstructive cholestasis [48]. FXR deletion protects hepatocytes by facilitating bile acid export into blood and renal excretion via a compensatory mechanism such as PXR activation. These findings suggest that protection of hepatocytes from cholestatic damage by FXR activation requires sufficient bile flow. FXR also induces the sinusoidal bile acid transporters OSTα and OSTβ [49], while PXR is involved in expression of MRP3 in mice [50]. FXR-null mice exhibit reduced fecal bile acid excretion as well as dysregulated bile acid synthesis [37]. Although FXR−RXR has been reported to bind to an everted repeat motif with 8-nucleotide spacer called ER-8 in the rat Mrp2 promoter [51], FXR deletion does not change Mrp2 expression in mice [52]. Multidrug resistance protein 3 MDR3 (ABCB4; corresponding to the murine MDR2), which is involved in phosphatidylcholine transport through the canalicular membrane, is induced by FXR through its binding to an IR1 element in the promoter [53, 54]. The cholesterol transporters ABCG5 and ABCG8 are also induced by cholic acid treatment of mice in an FXR-dependent manner [55].
FXR activation induces the bile acid transporter OSTα–OSTβ in the intestine and kidney [49] (Fig. 4.1). The intestinal intracellular bile acid-binding protein I-BABP is also a FXR target gene [25, 56]. IR1 elements have been identified in the promoters of these human genes [49, 56]. In contrast to the hepatic phenotype, FXR-null mice have efficient intestinal absorption of bile acids [52]. The physiological role of FXR in intestinal bile acid absorption remains to be elucidated.
Studies using pharmacological FXR activation and FXR-null mice have shown that FXR regulates triglyceride, cholesterol, and carbohydrate metabolism [4, 5, 32]. FXR deletion in mice has been reported to induce both increased and decreased glucose tolerance [57, 58]. FXR activation increases expression of genes involved in gluconeogenesis in hepatocytes [59], supporting the role of FXR in decreasing glucose tolerance. Recently, intestinal microflora have been found to play a role in energy metabolism through bile acid metabolism [16, 31]. Compared to conventionally raised mice, germ-free mice have decreased expression of the FXR targets Shp and Fgf15 in the distal ileum [16]. Germ-free mice have decreased secondary bile acids and increased TαMCA and TβMCA, which act as FXR antagonists, and show increased glucose tolerance, a similar phenotype to intestine-specific FXR-knockout mice [31]. Intestinal FXR-null mice are also resistant to diet-induced obesity. It is unknown how intestinal FXR antagonism or deletion induces metabolic effects. Bile acids have bacteriostatic effects and also protect the intestine from bacterial invasion through an FXR-dependent mechanism [60]. Thus, there are reciprocal interactions between host and bacteria through FXR regulation.
FXR exhibits immune modulatory action through a transrepression mechanism [61]. FXR agonist treatment attenuates inflammation in mouse models of hepatitis and colitis [61, 62]. The physiological relevance of bile acid in these FXR functions remains unclear. Elevated bile acid levels after partial hepatectomy accelerate liver regeneration in an FXR-dependent manner [63]. FXR activates the forkhead box M1 transcription factor, a cell cycle regulator controlling the G1/S and G2/M transitions, through binding to an IR0 element located in an intron of its gene [64]. The FGF15 signaling pathway induced by intestinal FXR activation is also involved in liver regeneration [65].
4.4 PXR, CAR, and VDR
PXR, CAR, and VDR belong to the NR1I nuclear receptor subfamily [1, 2]. PXR can respond to numerous structurally diverse compounds, including drugs, environmental contaminants, and bile acids, induces expression of transporters and enzymes involved in xenobiotic metabolism, and plays an important role in the detoxification and clearance of xenobiotics [4, 5, 66] (Fig. 4.1). PXR is activated by bile acids in potency order: 3-keto-LCA > LCA > DCA = CA [67, 68]. PXR activation represses expression of the bile acid synthetic genes, CYP7A1 and CYP8B1, without inducing SHP expression [69, 70]. PXR agonist treatment increases Cyp7a1 expression in wild-type mice but not in PXR-null mice [68]. PXR induces expression of human FGF19 and mouse Fgf15 genes [71], suggesting that FGF15/19 signaling plays a role in hepatic CYP7A1 suppression by PXR. PXR agonist treatment enhances bile acid detoxification by inducing the import transporter Oatp1a4 (Slco1a4; also called Oatp2), the detoxifying enzyme Cyp3a11, and the basolateral export transporter Mrp3 in the liver, leading to decreased serum bile acids and increased urinary bile acid excretion [50, 68, 72] (Fig. 4.2). CYP3A4, a human ortholog of mouse CYP3A11, is involved in the metabolism of 50–60% of pharmaceuticals as well as natural compounds such as steroids and herbal supplements [73]. CYP3A4 metabolizes LCA to 3-keto-LCA by 3-oxidation and hyodeoxycholic acid by 6α-hydroxylation [67, 74]. 3-Keto-LCA is a more potent PXR ligand than LCA, an interaction that enhances xenobiotic metabolism. PXR-binding elements have been identified in the CYP3A4 and OATP1B1 (SLCO1B1) promoters [75, 76]. Sulfation of LCA is mediated primarily by dehydroepiandrosterone sulfotransferase 2 (SULT2), which requires 3′-phosphoadenosine-5′-phosphosulfate (PAPS) as a donor molecule. The expression of Sult2 and PAPS synthase 2 (Papss2) is induced by PXR [77]. PXR is a candidate drug target for the treatment of cholestasis.
CAR is a nuclear receptor that regulates the transcription of genes involved in xenobiotic metabolism, cooperatively with PXR, and is abundantly expressed in the liver and intestine [78]. Although there is no evidence to date that endogenous bile acids are ligands for CAR, CAR has been shown to regulate bile acid metabolism (Fig. 4.1). CAR activation induces Cyp3a11 expression in PXR/FXR double knockout mice fed cholic acid [79]. Comparison of PXR-null mice, CAR-null mice, and PXR/CAR double knockout mice shows that CAR predominantly mediates induction of Cyp3a11 and Mrp3, while PXR is the major regulator of Oatp1a4 [80]. CAR is also involved in the induction of Sult2a, Papss2, and Mrp4 [81, 82]. In a bile duct ligation model of cholestasis, hepatic damage is increased in both PXR-null mice and CAR-null mice. PXR and CAR are required for elimination of toxic bile acids. Although these receptors have been reported to be involved in regulation of glucose and lipid metabolism [33], the role of bile acid signaling remains unknown.
VDR has been identified as a receptor for 1α,25-dihydroxyvitamin D3 (1,25(OH)2D3), the active form of vitamin D [33]. The secosteroid vitamin D3 is synthesized from 7-dehydrocholesterol through a photochemical reaction induced by sunlight exposure. Vitamin D3 is hydroxylated at the 25-position by CYP2R1 and CYP27A1 to yield 25-hydroxyvitamin D3 in the liver. CYP27A1 is a key enzyme in the alternate bile acid synthetic pathway and in side chain oxidation [9]. 25-Hydroxyvitamin D3 is further hydroxylated at the 1α-position by CYP27B1 to yield the active metabolite 1,25(OH)2D3 in the kidney [33]. The 1α-hydroxylation reaction is tightly regulated positively by parathyroid hormone and negatively by FGF23 and 1,25(OH)2D3. VDR activation induces feedback regulation by repressing CYP27B1 transcription through a poorly characterized mechanism. 1,25(OH)2D3 is also synthesized in extrarenal cells and tissues, such as macrophages. Dietary vitamin D2 and vitamin D3 are activated by the same processes, including hepatic 25-hydroxylation and renal 1α-hydroxylation. 1,25(OH)2D2 activates VDR as well as 1,25(OH)2D3 and has a different catabolic pathway than 1,25(OH)2D3. The VDR−RXR heterodimer binds preferentially to a two-hexanucleotide (AGGTCA or a related sequence) direct repeat motif separated by three nucleotides (DR3). Everted repeat elements separated by six, seven, eight, or nine nucleotides (ER6, ER7, ER8, or ER9) have also been identified as vitamin D response elements in genes including CYP3A4 [83, 84]. Pharmacological experiments using 1,25(OH)2D3 and its derivatives and animal studies using VDR-null mice have demonstrated that VDR regulates many physiological processes, including cellular growth and differentiation, hair cycle, immunity, cardiovascular function, lipid and xenobiotic metabolism, and neuronal function as well as bone and calcium metabolism [85, 86]. An understanding of VDR regulation of bile acid metabolism has been emerging since the discovery that VDR is activated by the secondary bile acid LCA and more effectively by 3-keto-LCA [87] (Fig. 4.1). Because CYP3A4 metabolizes LCA to 3-keto-LCA by 3-oxidation and hyodeoxycholic acid by 6α-hydroxylation [67, 74], LCA-activated VDR induces both enhanced VDR activation by 3-keto-LCA and detoxification to hyodeoxycholic acid, a mechanism similar to PXR (Fig. 4.2). Vitamin D signaling can induce this LCA detoxification mechanism [87]. Intestine-specific VDR knockout mice show increased contents of TCA and TDCA in the liver homogenate, a phenotype reversed by CYP3A4 transgene expression [88]. VDR also induces expression of other xenobiotic metabolism enzymes, such as MRP2, MRP3, MRP4, and SULT2A1 [89–91]. 1,25(OH)2D3 treatment does not induce hepatocyte target gene expression due to low VDR expression [92], suggesting a limited role of VDR in bile acid metabolism in the liver. Pharmacological activation of VDR enhances urinary excretion of bile acid by increasing the expression of bile acid transporters, such as MRP4, in the kidney [93] (Fig. 4.2) and does not alter bile acid accumulation in bile duct-ligated mice, but represses proinflammatory cytokine expression [94]. Interestingly, VDR ligand treatment represses hepatic Cyp7a1 expression in an FGF15-dependent mechanism, because Fgf15 is a VDR target gene [95]. In contrast, repeated administration of 1α-hydroxyvitamin D3, which is rapidly converted to 1,25(OH)2D3 in mice, increases Cyp7a1 expression [93]. This may be due to decreased bile acid pool sizes and subsequent relief from bile acid-induced suppression. Thus, VDR is involved in regulation of bile acid metabolism mainly in the intestine and kidney.
Apart from the direct effect on vitamin D absorption, a physiologic link connecting bile acids and calcium metabolism has not been demonstrated. LCA-derived ligands may exhibit selective non-calcemic VDR activity. Crystal structures of rat VDR with LCA and its derivatives show that they bind to the VDR−LBP with an orientation opposite to that of 1,25(OH)2D3 in both horizontal and vertical planes [33, 96]. The side chain carboxyl group is directed toward the β-turn side, the A ring faces helix 12, and the β-face of the steroid is directed toward helix 7 in the bottom of the ligand-binding pocket. The crystal structure of zebrafish VDR with LCA reveals the binding of two LCA molecules [97]. While one LCA binds to the canonical LBP, the second one is anchored to a site located on the VDR surface and is suggested to promote stabilization of the active conformation. LCA acetate and LCA propionate are more potent VDR agonists than LCA and can induce differentiation of myeloid leukemia cells, a useful cellular assay for pharmacological effects of 1,25(OH)2D3 and its derivatives [98, 99]. Administration of LCA acetate or LCA propionate to mice effectively induces tissue VDR activation without causing hypercalcemia [99]. Intestine-specific VDR deletion induces a change in the intestinal microbial flora and increases susceptibility to colitis in a mouse model of inflammatory bowel disease [100]. LCA can substitute for vitamin D in elevating serum calcium levels, mobilizing calcium from bone, and inducing expression in the kidney of the VDR target gene CYP24A1 only in vitamin D-deficient rats [101]. The VDRs of non-mammalian species, such as lamprey, zebrafish, and Xenopus laevis, are insensitive to bile acids and bile alcohols [13]. The ability of VDR to respond to LCA may be a more recent evolutionary development, occurring after appearance of the synthetic pathway of CDCA in hepatocytes and its conversion to LCA in bacteria. LCA may be involved in selective VDR functions, such as regulation of intestinal microbial colonization.
4.5 Other Receptors
UDCA is used in the treatment of cholestatic disease, such as primary biliary cirrhosis [102]. Glucocorticoid receptor (GR; NR3C1) responds to UDCA in a ligand-independent way [103]. Although it remains unclear whether GR mediates the pharmacological action of UDCA, the combination of UDCA and a FXR or PXR activator may be useful in the treatment of cholestasis. Bile acid-activated G protein-coupled receptors, such as TGR5 and sphingosine-1-phosphate receptor 2, have also been identified [4]. Elucidation of the bile acid signaling network should provide insight into pathophysiology of metabolic diseases and development of novel therapeutics.
4.6 Conclusion
Bile acids are important not only as bile components for lipid digestion and absorption but also as signaling molecules for nuclear receptors, such as FXR, PXR, and VDR. Bile acids regulate bile acid and lipid metabolism through activation of these receptors and also exhibit other physiological actions. Intestinal bacteria metabolize bile acids and can influence host metabolism by changing nuclear receptor activities. Bile acids might represent “prototypes” of steroid hormones and also serve as signaling molecules for communication between host and bacteria. The bile acid receptors FXR, PXR, and VDR are promising drug targets for metabolic diseases.
Acknowledgments
The author thanks Dr. Andrew I. Shulman for editorial assistance.
References
1.
Evans RM, Mangelsdorf DJ. Nuclear receptors, RXR, and the big bang. Cell. 2014;157(1):255–66. doi:10.1016/j.cell.2014.03.012.PubMedPubMedCentral
2.
Makishima M. Nuclear receptors as targets for drug development: regulation of cholesterol and bile acid metabolism by nuclear receptors. J Pharmacol Sci. 2005;97(2):177–83. doi:10.1254/jphs.FMJ04008X4.PubMed
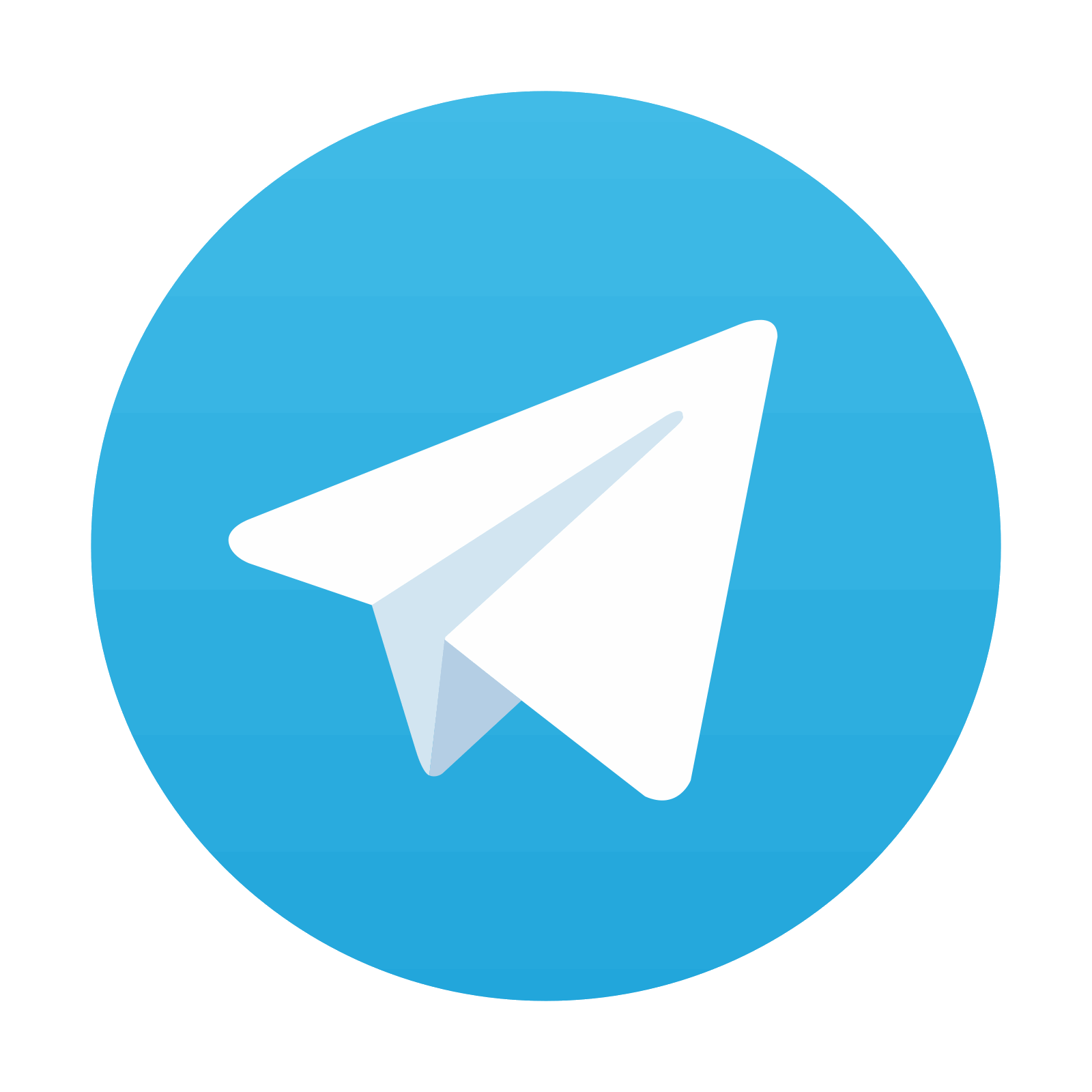
Stay updated, free articles. Join our Telegram channel
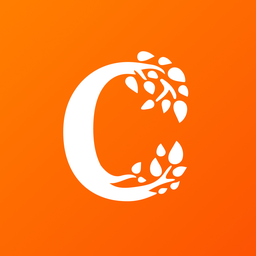
Full access? Get Clinical Tree
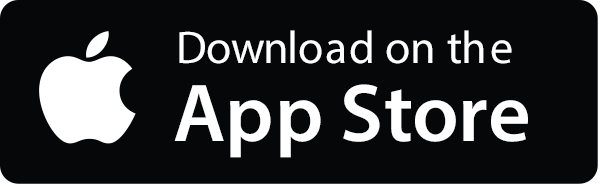
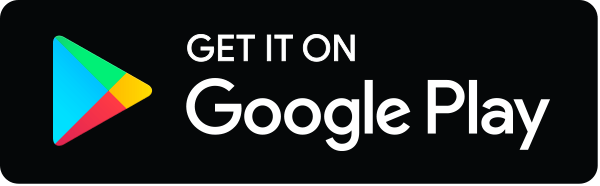