Pancreatic ductal adenocarcinoma comprises more than 90% of pancreatic tumors. It is the 10th most common cancer diagnosis in the United States, but represents the fourth most common cause of cancer deaths, with nearly 40,000 deaths reported in 2010. This disproportionate mortality rate highlights the aggressive and deadly nature of this disease. Unfortunately, despite improvements in mortality rates in other malignancies, little improvement has been observed in pancreatic adenocarcinoma.
Surgery, most often pancreatoduodenectomy, remains the standard of care and offers the only chance of cure for pancreatic cancer. Unfortunately, only 20% of patients are found to be acceptable surgical candidates at the time of diagnosis. Radiation therapy can be utilized in a wide range of clinical presentations of pancreatic cancer, from early stage to widely metastatic disease, and its role is evolving, along with surgery and chemotherapy, aided by advances in technology and technique.
Radiotherapy of pancreatic cancer is technically challenging for a variety of reasons. Pancreatic tumors are often difficult to clearly define, even with advanced imaging techniques like fluorodeoxyglucose positron emission tomography ( 18 F-FDG-PET) and magnetic resonance imaging (MRI), making clear designation of treatment volumes difficult. In addition, organ motion throughout the respiratory cycle can further compromise the accuracy of planned treatment delivery. Also, the proximity of radiosensitive organs, such as the bowel, liver, and kidney, to the pancreas, can result in significant normal-tissue toxicity with the delivery of tumoricidal doses of radiation outside of the target. Recent technologic advances in the planning and delivery of radiation therapy, such as intensity-modulated radiation therapy (IMRT), arc therapy, image-guided radiation therapy (IGRT), and stereotactic ablative body radiotherapy (SABR), allow the potential for greater tumor control with reduced treatment-related toxicity and represent significant steps forward in the field.
Radiotherapy in the Treatment of Pancreatic Adenocarcinoma
Although surgery is the standard treatment that offers the only reasonable chance of cure, 5-year survival rates for patients who undergo a tumor-free margin (R0) resection are only 10% to 25%, which emphasizes the importance of adjuvant treatment. There also remains the problem of how to optimally manage inoperable patients, especially those without evidence of distant spread. Unfortunately, the median survival for patients who are unable to undergo resection is on the order of 10 to12 months, highlighting the need for more effective therapeutic strategies in this population. The indications for the use of radiotherapy in the treatment of pancreatic cancer are not straightforward. It has been suggested that preoperative (neoadjuvant) radiotherapy treatment may provide some benefit by improving outcomes and potentially converting inoperable cases to operable ones.
Unlike with other gastrointestinal (GI) malignancies, randomized trials have not led to clear indications for the use of adjuvant chemoradiation for pancreatic cancer. Early trials from the United States found a survival benefit, whereas European trials found little or no benefit to chemoradiation but a significant benefit with the use of adjuvant chemotherapy alone. All of these early trials had severe limitations, and their radiation doses and delivery have been deemed ineffective by today’s standards. As a consequence, the role of radiation therapy still remains controversial. Currently, a combined Radiation Therapy Oncology Group (RTOG)/European Organisation for Research and Treatment of Cancer (EORTC) trial (RTOG 0848) is testing the small-molecule kinase-inhibitor erlotinib in combination with gemcitabine and the role of adjuvant chemoradiation using modern doses (50.4 Gy), modern scheduling, and modern radiation techniques.
Preoperative radiotherapy has been shown to be both feasible and effective in GI malignancies like rectal and esophageal cancer. Its use is appealing in the treatment of pancreatic cancer for the following reasons: (1) it may increase the chances of an R0 resection; (2) it may allow for the downstaging of inoperable disease, thereby leading to surgical resection and a chance for cure; (3) it allows for earlier initiation of systemic therapy, which may provide a theoretical advantage in the treatment of distant disease when the burden is low rather than 6 to 8 weeks postoperatively ; (4) it may spare patients an unnecessary surgery if metastatic disease is detected during the course of preoperative treatment; and (5) it increases the efficacy of radiation and chemotherapy when given with an intact vascular supply to the tumor, making it easier to identify the target.
Target Definition and Image-Guided Radiation Therapy
Historically, radiation therapy has been guided by imaging, but a more recent collection of research and advances in technology have vastly improved our understanding and ability to utilize imaging throughout the entire treatment process, a concept that is broadly termed image-guided radiation therapy (IGRT). To understand the power of IGRT, one must understand how the treatment area is defined.
Conventional nomenclature refers to the visible tumor as the gross tumor volume. An internal tumor volume has been defined as the volume occupied by the tumor throughout the respiratory cycle to account for motion associated with diaphragmatic excursion. The area at risk for clinical spread of tumor, such as the draining lymph nodes and tissue adjacent to the tumor, which may have been microscopically invaded, is called the clinical treatment volume. Typically, a fourth volume, the planning treatment volume, has also been defined, which is an additional expansion to account for inaccuracies and variability in daily patient positioning. The exact amount of the expansion depends on the reproducibility of the patient positioning and the quality and frequency of imaging to verify proper alignment. The more accurately one can position the patient and confidently deliver the treatment, the less expansion required, resulting in a smaller overall volume of nearby normal tissue that is treated, which reduces treatment-related toxicity.
Multiple imaging modalities are used to diagnose and stage pancreatic cancer, many of which can assist in the delineation of these volumes, including endoscopic ultrasound, endoscopic retrograde cholangiopancreatography, magnetic resonance imaging (MRI), computed tomography (CT), and positron emission tomography (PET). Each of these imaging modalities has its limitations, such as significant interobserver variability, but altogether they provide the treating radiation oncologist with valuable information about location, size, extent of spread, and possible lymph node involvement in each individual.
Modern radiation treatment planning begins by obtaining a high-quality CT scan used to contour the tumor as well as surrounding normal tissue and organs. Even with the use of intravenous contrast, pancreatic tumors can be isodense and difficult to distinguish from surrounding normal tissue. For example, one study comparing the size of pancreatic tumors as seen on CT and the actual size of surgical specimens after resection found that the CT scan significantly undersized pancreatic tumors by a median 7 mm in most patients (84%).
MRI provides excellent soft-tissue definition, but image quality is degraded by movement. Although motion artifact-reducing MRI scanners do exist, most radiation therapy departments are not equipped with them.
18 F-FDG-PET provides the ability to distinguish metabolically active disease and is commonly being utilized during radiotherapy simulation in an increasing number of cancer types ( Fig. 1 ). Pancreatic tumors are generally 18 F-FDG avid, although the avidity can vary, and cystic tumors can result in false positivity.

At some institutions, PET and CT are obtained at the time of treatment planning for pancreatic tumors. It has been reported that PET and CT may be particularly useful in distinguishing pancreatic head tumors from the adjacent duodenum, which is particularly important, as radiation dose to this area is a critical determination of toxicity during SABR treatments. Additionally, the degree of PET positivity in pretreatment scans has been found to hold prognostic value, as patients with higher maximum standard uptake values displayed worse progression-free and overall survival rates.
Tumors in the thorax and upper abdomen move throughout the respiratory cycle. It has been reported that pancreatic tumors can move as much as 2 to 3 cm with normal respiration. Four-dimensional CT (4DCT) was developed to address the problem of organ motion during respiration. 4DCT is a type of CT scan commonly used for planning radiation treatment and creates a CT “movie” to determine how tumors move during breathing. 4DCT image processing tools are readily available and allow the determination of tumor motion extent and the design of motion-compensating treatment strategies. The main goal of 4DCT imaging is to design treatment strategies that safely reduce treatment margins, thus, sparing healthy tissue. Among these strategies is airway breathing control, which is when a patient holds his or her breath during treatment delivery to eliminate motion. With respiratory gating, radiation is delivered only during specific phases of the patients’ respiratory cycle. Abdominal compression devices can limit the amount of diaphragmatic excursion, reducing organ motion during breathing. And with tumor tracking, the radiation beam follows, or tracks, the tumor as it moves during the respiratory cycle.
Target Definition and Image-Guided Radiation Therapy
Historically, radiation therapy has been guided by imaging, but a more recent collection of research and advances in technology have vastly improved our understanding and ability to utilize imaging throughout the entire treatment process, a concept that is broadly termed image-guided radiation therapy (IGRT). To understand the power of IGRT, one must understand how the treatment area is defined.
Conventional nomenclature refers to the visible tumor as the gross tumor volume. An internal tumor volume has been defined as the volume occupied by the tumor throughout the respiratory cycle to account for motion associated with diaphragmatic excursion. The area at risk for clinical spread of tumor, such as the draining lymph nodes and tissue adjacent to the tumor, which may have been microscopically invaded, is called the clinical treatment volume. Typically, a fourth volume, the planning treatment volume, has also been defined, which is an additional expansion to account for inaccuracies and variability in daily patient positioning. The exact amount of the expansion depends on the reproducibility of the patient positioning and the quality and frequency of imaging to verify proper alignment. The more accurately one can position the patient and confidently deliver the treatment, the less expansion required, resulting in a smaller overall volume of nearby normal tissue that is treated, which reduces treatment-related toxicity.
Multiple imaging modalities are used to diagnose and stage pancreatic cancer, many of which can assist in the delineation of these volumes, including endoscopic ultrasound, endoscopic retrograde cholangiopancreatography, magnetic resonance imaging (MRI), computed tomography (CT), and positron emission tomography (PET). Each of these imaging modalities has its limitations, such as significant interobserver variability, but altogether they provide the treating radiation oncologist with valuable information about location, size, extent of spread, and possible lymph node involvement in each individual.
Modern radiation treatment planning begins by obtaining a high-quality CT scan used to contour the tumor as well as surrounding normal tissue and organs. Even with the use of intravenous contrast, pancreatic tumors can be isodense and difficult to distinguish from surrounding normal tissue. For example, one study comparing the size of pancreatic tumors as seen on CT and the actual size of surgical specimens after resection found that the CT scan significantly undersized pancreatic tumors by a median 7 mm in most patients (84%).
MRI provides excellent soft-tissue definition, but image quality is degraded by movement. Although motion artifact-reducing MRI scanners do exist, most radiation therapy departments are not equipped with them.
18 F-FDG-PET provides the ability to distinguish metabolically active disease and is commonly being utilized during radiotherapy simulation in an increasing number of cancer types ( Fig. 1 ). Pancreatic tumors are generally 18 F-FDG avid, although the avidity can vary, and cystic tumors can result in false positivity.
At some institutions, PET and CT are obtained at the time of treatment planning for pancreatic tumors. It has been reported that PET and CT may be particularly useful in distinguishing pancreatic head tumors from the adjacent duodenum, which is particularly important, as radiation dose to this area is a critical determination of toxicity during SABR treatments. Additionally, the degree of PET positivity in pretreatment scans has been found to hold prognostic value, as patients with higher maximum standard uptake values displayed worse progression-free and overall survival rates.
Tumors in the thorax and upper abdomen move throughout the respiratory cycle. It has been reported that pancreatic tumors can move as much as 2 to 3 cm with normal respiration. Four-dimensional CT (4DCT) was developed to address the problem of organ motion during respiration. 4DCT is a type of CT scan commonly used for planning radiation treatment and creates a CT “movie” to determine how tumors move during breathing. 4DCT image processing tools are readily available and allow the determination of tumor motion extent and the design of motion-compensating treatment strategies. The main goal of 4DCT imaging is to design treatment strategies that safely reduce treatment margins, thus, sparing healthy tissue. Among these strategies is airway breathing control, which is when a patient holds his or her breath during treatment delivery to eliminate motion. With respiratory gating, radiation is delivered only during specific phases of the patients’ respiratory cycle. Abdominal compression devices can limit the amount of diaphragmatic excursion, reducing organ motion during breathing. And with tumor tracking, the radiation beam follows, or tracks, the tumor as it moves during the respiratory cycle.
Image Guidance During Treatment Delivery
Although knowing and accounting for tumor and organ motion during the planning phase of radiotherapy is critical, this information must also be recalled during actual treatment delivery. Mechanical methods (airway breathing control, respiratory-gating, and abdominal compression) that limit motion at the time of simulation and planning are also used during the daily treatment delivery.
Verification of setup positioning is critical to ensuring accurate radiation delivery to the target. Traditionally, verification was done with megavoltage (MV) imaging using the linear accelerator (linac) as a beam source and a detector mounted at the opposite side of the linac. The quality of MV images of the patient in the actual treatment position varies, and the images are mainly used to confirm that the bony anatomy of the patient is well aligned with respect to a reference image used during treatment planning. However, with more-sophisticated radiation treatment delivery techniques available, IGRT was developed to improve upon accuracy. Modern linacs now have a kilovoltage (kV) source with a flat-panel detector mounted orthogonally to the treatment beam, which improves resolution imaging for bony-anatomy alignment. Cone-beam CT (CBCT), a method similar to conventional 3-dimensional anatomic imaging, can be performed to align patients based on soft tissue, which is otherwise impossible with MV or kV 2-dimensional imaging. Whether used alone or combined, either method will ensure accurate patient setup when a high degree of accuracy is needed.
One of the difficulties particular to treatment of the pancreas with IGRT is that bony anatomy does not necessarily reflect the position of the tumor. The farther the tumor is from the bony landmarks used to localize it radiographically, the greater the possibility for error. To compensate for this distance, gold fiducial markers are placed in or as near to the tumor as possible because they can be easily seen on x-ray imaging. Park and colleagues describe a technique by which gold fiducials are placed via fine-needle aspiration under endoscopic ultrasound. Revealing only a 5% complication rate, the procedure was found to be safe and effective with a median of 5 implantations and a mean of 3 fiducials seen at the time of CT simulation, which was secondary to fiducial loss or migration. Percutaneous fiducial placement has also been reported.
These many imaging advances, used alone or together, can decrease uncertainty when defining treatment volumes and increase accuracy when irradiating these volumes. Delivering radiation with better conformality allows physicians to safely treat smaller volumes to higher doses, a paradigm that is reaching its apotheosis in SABR.
Intensity-Modulated Radiotherapy
IMRT is a highly conformal radiation delivery method that uses a device known as the multileaf collimator (MLC) to dynamically shape radiation beams into varying patterns from multiple beam angles. IMRT represents an increase in complexity over 3-dimensional conformal radiotherapy where the modulation of the beam intensity is limited. In IMRT, more than 4 complex radiation fields are divided into dozens of smaller beamlets, each with an intensity that can be modulated by moving the MLC to achieve the desired dose distribution across the target volume. The main advantage of IMRT is that it provides more precise control over the target as well as normal-tissue dose distribution. The selection of the beamlets and their individual intensity modulations are determined by computer-based iterative optimization algorithms that satisfy dose limits to defined normal structures and provide adequate radiation delivery to the target.
IMRT allows highly precise gradients of dose to be deposited within tissue, for example, creating a sharp “drop off” of dose from the edge of a tumor to a critical nearby structure ( Fig. 2 ). While IMRT allows for radiation doses to be delivered more precisely, it does not eliminate dose to normal tissues. In fact, it is often the case that a larger volume of at-risk tissue is irradiated using IMRT, albeit at a lower dose (also termed a low-dose bath ). Whether this pattern of normal-tissue dosing has a similar threshold and manner of toxicity as that seen with older forms of treatment and how organs differ in their sensitivity to this type of irradiation are topics of ongoing clinical investigation.
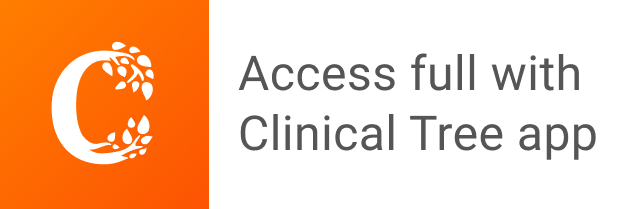