Inherited mitochondrial disorders of fatty acid oxidation (FAO) and the mitochondrial respiratory chain both affect mitochondrial oxidative phosphorylation. The respiratory chain is made up of five separate complexes in the inner mitochondrial membrane. The chain generates ATP from oxidation of NADH in all tissues. Disruption of this chain has major consequences, especially in organs with the highest demand for energy: muscle, brain, liver, heart, and kidney. As a generalization, FAO disorders tend to present acutely, often in association with intercurrent illness and, with prompt recognition, recover. Disorders of mitochondrial respiratory chain, however, tend to be progressive, with incomplete recovery between episodes. With time, more organ systems are involved, and, in particular, neurologic involvement is frequent. This chapter concentrates on the hepatic involvement in these diseases rather than a comprehensive review of these fascinating disorders.
Defects in Fatty Acid Oxidation
Fatty acid oxidation provides an important source of energy during fasting, especially in childhood when glycogen stores are limited. Hepatic FAO produces ketone bodies, which are an important secondary energy source for many tissues, including the brain. Defects in any of the proteins in this pathway may lead to disease, and more than 20 individual defects have been recognized to date. The combined incidence of all FAO disorders is approximately 1/9000 live births, with a wide ethnic variation in the distribution of specific defects. All have autosomal recessive inheritance. The principles of pathophysiology, diagnosis, and treatment will be discussed.
Pathophysiology
The first step in fatty acid metabolism is lipolysis in response to fasting, resulting in circulating free fatty acids (FFAs). FFAs are then transported across the plasma membrane and are transformed to coenzyme A (CoA) esters in the cytosol before entry to the mitochondria by the carnitine cycle for further metabolism. Long-chain acyl-CoA esters must first be conjugated to carnitine before active transport across the mitochondrial membrane, and once in the mitochondria then are retransformed into acyl-CoA esters. The carnitine shuttle results in export of the freed carnitine moiety to maintain the cytoplasmic pool. Carnitine is an essential nutrient in the newborn period but is sufficiently supplied in breast and formula feed. Low carnitine levels imply increased losses, usually urinary, which is the mechanism in carnitine transporter deficiency. Defects have been recognized in each of these steps and in carnitine uptake.
Within the mitochondria, acyl-CoA esters undergo β-oxidation. This is a four-step, cyclical process where fatty acids are sequentially degraded to acetyl-CoA, with a molecule of acetyl-CoA being released at each step. β-Oxidation results in a continuous flow of electrons to the electron transport chain by electron transfer flavoprotein (ETF) and its dehydrogenase (ETF-DH). The first two cycles of β-oxidation of long-chain fatty acids take place at the inner mitochondrial membrane using very long-chain acyl-CoA dehydrogenase (VLCAD) and the associated mitochondrial trifunctional protein (MTP). This complex contains the other three enzymes needed to complete a cycle, including long-chain 3-hydroxy-acyl-CoA dehydrogenase (LCHAD).
Medium- and short-chain fatty acids can cross the mitochondrial membrane independent of carnitine or long-chain transporters, which provides the rationale for the use of medium-chain triglyceride (MCT) in long-chain defects. Whether of dietary origin or a product of β-oxidation, these are then oxidized within the mitochondrial matrix by length-specific enzymes.
Within the liver, acetyl-CoA is used for ketone body production. Once lipolysis occurs, there should be accompanying ketone production. Hence, finding significant FFAs with a disproportionally low 3-OH butyrate level is a useful diagnostic clue.
Defects at any stage in the FAO pathway will result in local failure of energy production, inadequate ketone body production, and hypoglycemia. Hypoglycemia results from both reduced hepatic glucose production and increased peripheral consumption. When β-oxidation is defective, abnormal and potentially toxic metabolites may accumulate. In addition, some specific mutations may result in the production of a structurally abnormal protein, which may become misfolded with consequent intracellular disruption and stress.
Depending on the site of the block, differing acylcarnitines will accumulate proximally, and their pattern detection in blood and urine can help to localize the defect. Similarly, differing urinary organic acid profiles may help characterize the site of the block. FFA may undergo ω-oxidation in microsomes, thereby producing dicarboxylic acids. Some of these metabolic signatures may be manifest only at the time of metabolic instability, whereas in some defects they can be persistent.
Clinical Features and Diagnosis
The most common presentation is an acute hepatic phenotype infancy with hypoketotic hypoglycemia and encephalopathy. This may be provoked by weaning or intercurrent infection. Hepatomegaly, elevated transaminases, and modest hyperammonemia occur in more than 80% of cases, with cholestasis in up to one-third. In severe cases, there may be a fully fledged “Reyes syndrome like” illness, which can be fatal. Neurologic, cardiac, and muscular symptoms are common. A summary of clinical and biochemical features is shown in Table 71-1 .
Defect | Clinical Phenotype | Other Features | Metabolic Abnormalities | Diagnosis Confirmation |
---|---|---|---|---|
CTD | H, C, M | Low free carnitine | EM | |
CPT1 | H, C, R, M | RTA | High free carnitine | EM |
CACT | H, C, M | Early death | C16 and 18 species | EM |
CPT2 | H | RTA | C16 and 18 | 493C>T |
VLCAD | H, C,R, M | C14, 16, and 18 | ||
MCAD | H, C,R, M | C8 DCA | 985A>G in 90% symptomatic cases | |
LCHAD/MTP | H | Retinopathy, Neuropathy | C16 and 18 species DCA | 1528G>C |
SCHAD | H | Hyperinsulinism | C4-OH Urinary ethylmalonic acid | EM |
MAD | H, C, M | Congenital malformations, Renal cysts, RTA | C6, 8,10, and 12 Urinary ethylmalonic, glutaric, and adipic acids DCA | EM |
True acute liver failure is uncommon but has been described in LCHAD, Acyl-CoA- Dehydrogenase deficiency (ACAD9), multiple acyl-CoA dehydrogenase MAD, and carnitine/acylcarnitine translocase deficiency.
Other clinical presentations in children include a cardiac phenotype with infantile cardiac dysfunction or arrhythmia and a myopathic phenotype in later childhood characterized by muscle pain, limited exercise tolerance, and recurrent rhabdomyolysis. Sadly, sudden infant death can be the first presentation.
A fascinating aspect of this group of disorders is the association with maternal illness during pregnancy. This association was first noted when a mother with acute fatty liver of pregnancy (AFLP) delivered an infant with LCHAD deficiency. Recently it has become clear that this association is not limited to fetal LCHAD deficiency but may occur in any FAO defect including medium- and short-chain defects. The maternal illness may be part of a spectrum ranging from AFLP to the HELLP syndrome (hemolysis, elevated liver enzymes, and low platelets). The mechanism of the maternal illness is unclear but presumably results from limited ability of the heterozygote maternal liver to detoxify metabolites produced by the fetus in combination with the metabolic stress of pregnancy. Efforts to detect metabolic abnormalities during affected pregnancies have been largely uninformative, but recently long-chain hydroxyacyl carnitine species were detected in a carrier of LCHAD deficiency at 31 weeks of pregnancy during AFLP, supporting the concept of metabolic intoxication. The incidence of AFLP in pregnancies where the fetus has a FAO defect is significant, but the converse is not true. At most, FAO disorders account for 20% of AFLP and probably less. Given the implications for the child and future pregnancies it is still crucial that all children born after pregnancy affected by AFLP are systemically screened for FAO disorders, and if necessary prospectively treated until results are available.
With the advent of newborn screening, a broader category of milder and even asymptomatic phenotypes is increasingly recognized.
Diagnosis
The best diagnostic yield will be from blood acylcarnitine profile and urinary organic analysis at the time of metabolic instability. It is important that all institutions caring for children have established protocols for the structured investigations of acutely unwell children with hypoglycemia.
Typical biochemical investigations reveal:
- •
hypoketotic hypoglycemia
- •
elevated transaminases
- •
low plasma carnitine and abnormal acyl carnitine profile
- •
elevated plasma free fatty acids/3-hydroxybutyrate ratio
- •
increased urinary dicarboxylic acids
- •
mild to moderate hyperammonemia and hyperlactic acidemia
- •
moderate hyperuricemia
- •
elevated plasma creatinine kinase
Confirmation of the individual defect may be difficult due to the variety of potential enzyme defects. In selected patients, loading tests with medium-chain or long-chain triglyceride may be helpful.
In medium-chain acyl-CoA dehydrogenase deficiency and LCHAD, common single mutations make the diagnosis simpler. In addition, common mutations causing Carnitine palmitoyl transferase types 1 (CPT1) & 2 (CPT2) and short-chain acyl-CoA dehydrogenase deficiency (SCAD) make a genetic approach to diagnosis practical. In VLCAD, the c.243V>A mutation is associated with a mild phenotype.
All characterized defects are expressed and can be assayed in skin fibroblasts. In vitro analysis with labeled myristate, palmitate, and oleate are useful for diagnostic screening, and in vitro acylcarnitine profiling can provide specific diagnostic information.
There remains a group of patients who have a phenotype suggestive of FAO, but in whom no specific defect can be demonstrated. Notwithstanding that, there may yet be unrecognized pathways; the concept of synergistic heterozygosity has been proposed, where two heterozygous defects at different steps in FAO might result in functional effects. A similar mechanism could account for some of the significant phenotypic variability seen even in recognized defects.
Fatty Acid Transport Defects
The mechanism for transport of long-chain fatty acids into the cell remains uncertain. Two children with liver failure who had defective fatty acid uptake have been described. Although their illness had many other features of FAO disorders, there was conspicuously little steatosis. Both underwent successful liver transplantation and no further cases have been described.
Carnitine Transporter Deficiency
The usual presentation of carnitine transporter deficiency is with heart failure accompanied by muscular weakness in preschool children. Acute hepatic presentation is rare. Very low free and total carnitine levels are diagnostic. Treatment with lifelong oral carnitine is life-saving.
Carnitine Palmitoyl Transferase 1 Deficiency
Carnitine palmitoyl transferase 1 (CPT1) deficiency is a disorder caused by a defect in the carnitine palmitoyl transferase enzyme at the outer mitochondrial membrane. This defect prevents mitochondrial uptake of long-chain fatty acyl-CoA, the rate-limiting step in fat oxidation. The highest incidence is in the Inuit population, where it is associated with a variant in the CPT1A gene, which may be pathogenic. Presentation is usually in infancy with acute hypoketotic hypoglycemia and occasionally with cholestasis.
Investigations demonstrate the typical features of FAD except:
- •
plasma carnitine may be normal or increased,
- •
acylcarnitine profile is normal, and
- •
muscle biopsy may show accumulation of glycogen and lipid.
The prognosis is good, and normal growth and development can be achieved.
Multiple Acyl-coenzyme: A Dehydrogenase Defect (Glutaric Acidemia Type 2)
This rare disease is due to deficiency of either the α or β chain of electron transfer flavoprotein (ETF) or of its dehydrogenase (ETF-DH), which prevents energy production by all acyl-CoA dehydrogenases. This is a heterogenous disorder with a number of phenotypes including:
- •
severe neonatal illness with profound metabolic acidosis
- •
congenital malformations, including polycystic kidneys, defects in the anterior abdominal wall and genital abnormalities
- •
dysmorphic features, including low-set ears, high forehead, rocker-bottom feet, single palmar creases
- •
later myopathic presentation, which may be associated with spasticity or extrapyramidal features
- •
- •
increased urinary organic acids, including ethylmalonic, glutaric, and adipic acids
- •
usually elevated plasma lactate
Patients who survive or present later should be assessed for riboflavin responsiveness, and if responsive, treated with 100 to 300 mg per day riboflavin. Synthetic ketone bodies have been used successfully in three children, with significant improvement in muscle and cardiac function.
Many infants with structural abnormalities die within the first week of life. Some respond to initial treatment but often succumb to cardiomyopathy in infancy.
Very-long-chain Acyl-CoA Dehydrogenase (VLCAD) Deficiency
In very-long-chain acyl-CoA dehydrogenase (VLCAD) deficiency, the most common presentation is a late-onset muscular phenotype. Rarely, more severely affected patients may present with cardiomyopathy in infancy. Universal neonatal screening has shown that the majority of patients remain asymptomatic, especially those with the c.243V>A mutation.
Long-chain 3-hydroxyacyl-CoA Dehydrogenase (LCHAD) and Mitochondrial Trifunctional Protein (MTP) Deficiencies
The enzyme, long-chain 3-hydroxyacyl-CoA dehydrogenase (LCHAD), is a component of the MTP complex of the inner mitochondrial membrane. These enzymes have optimal activity for C 12 to C 16 chain length fatty acids. Deficiencies of both isolated LCHAD and of the whole MTP complex occur. A common mutation, c.1528G>C, has been recognized, accounting for 70% of abnormal alleles in isolated LCHAD.
Isolated LCHAD usually has an infantile hepatic presentation. A small proportion of patients develop cholestasis and progressive liver disease. Cardiomyopathy is not uncommon. With time, myopathic features predominate and pigmentary retinopathy develops. MTP deficiency is more variable, ranging from a fatal neonatal presentation with cardiomyopathy to a myopathic presentation in adulthood. Maternal acute fatty liver of pregnancy may develop when a heterozygote mother carries a fetus with LCHAD deficiency, particularly but not exclusively if the fetus carries the c.1528G>C mutation.
Diagnosis is confirmed either by mutation detection or by specific enzymatic diagnosis from cultured fibroblasts.
Medium-chain Acyl-CoA Dehydrogenase (MCAD) Deficiency
MCAD deficiency is by some distance the most common fatty acid deficiency (FAD) in Caucasians. The incidence in the United Kingdom is approximately one per 10,000, with a single mutation (c.985A>G) accounting for 90% of abnormal alleles in symptomatic cases. The presentation is usually a hepatic phenotype in infancy, but asymptomatic cases are common, especially since the advent of newborn screening. De novo presentation in later childhood or as an adult is rare but can be fatal.
Universal newborn screening was introduced in the United Kingdom in 2009. Of interest, another mutation (c.199C>T) is more common in screened subjects. Historically, up to 25% died of their acute illness, with some survivors having neurologic sequelae. Dietary treatment is unnecessary, but children should avoid MCT. Standard management by avoiding unnecessary fasting and use of an “emergency regimen” results in an excellent outlook.
Acyl-CoA Dehydrogenase 9 (ACAD) Deficiency
ACAD9 has a substrate spectrum similar to that of VLCAD, but in vivo its main role appears to be in assembly of complex 1 of the respiratory chain. Presentations have included acute liver failure, recurrent Reye-like syndrome, and cardiomyopathy. Investigations have shown prominent C18:1 and C18:2 carnitine species, but in other cases were normal.
Treatment of FAO Disorders
The primary aim of treatment is to avoid excess fasting and suppress lipolysis, especially in the first 6 months of life. This may require the occasional use of nasogastric tube feeds or nocturnal uncooked cornstarch, but in general, these should not be necessary. All children should be provided with written individualized emergency regimens for use during intercurrent illnesses.
In disorders of long-chain fat oxidation, dietary LCT should be restricted with MCT supplementation. Essential fatty acid supplementation should be provided with walnut oil.
LCT restriction can be relatively modest in MAD and VLCAD (25% to 30% of calorie intake).
In LCHAD/MTP, LCT intake should be as low as possible. This prevents the use of breast milk and requires use of specific MCT-based formula feed. Even after weaning, LCT intake should be kept to less than 10% of calories. Docosahexanoic acid supplementation is recommended; although it does not prevent eventual progression of retinopathy, its use is associated with retention of retinal function and visual acuity.
All patients with muscle symptoms should be encouraged to maintain activity levels with the use of a preexercise MCT supplement where necessary.
Routine carnitine supplementation is no longer recommended except for carnitine transporter deficiency and in some children with MAD.
Bezafibrate has been shown in vitro to stimulate muscle cell oxidation by increasing gene expression of CPT2 and VLCAD. An uncontrolled study suggested that it had useful clinical effects in patients with CPT2 deficiency, but this was not confirmed in a cross-over study in patients with CPT2 and VLCAD. Hence, at present this can only be seen as an investigational agent.
A distinction needs to be made between those who present clinically and those who are detected by newborn screening. In VLCAD, it is reasonable to continue with breast-feeding if creatinine kinase level is normal. If there is evidence of myopathy, then LCT restriction should be introduced. LCHAD should be treated aggressively from diagnosis, irrespective of mode of presentation. A suggested monitoring strategy is summarized in Box 71-1 .
The general review schedule should be every 3 months in the first year and subsequently, annually. However, patients with long-chain defects will usually require more frequent visits, even after infancy.
Each Visit
Growth and clinical assessment for developmental status, motor development, cardiac, and abdominal examination.
Annual
Hepatic transaminases, creatine kinase, and acylcarnitines.
If on MCT diet, nutritional profile, red cell fatty acids, and fat-soluble vitamin levels.
Long-chain defect, abdominal ultrasound and echocardiography.
Trifunctional protein defect, ophthalmologic assessment, and nerve conduction velocity.
Outcome
The prognosis for those who present clinically is poor, especially if they present in the neonatal period. Overall mortality in the first episode is as high as 60%, whereas once a patient has been diagnosed and their family provided with strategies, the risk of future decompensation is low. A comprehensive national study has shown an overall mortality of 50%, with 60% mortality in LCHAD and VLCAD deficiency where the presentation is clinical. Twenty percent to 30% of those who present clinically with MCAD and survive are left with significant developmental sequelae.
In contrast, the outlook following diagnosis by newborn screening is much more encouraging. Mortality and morbidity from MCAD, LCHAD, and VLCAD have decreased in screened population, albeit at the cost of recognizing many cases that would have remained asymptomatic. The impact of screening on rarer disorders is still uncertain, but appears generally positive.
Disorders of the Mitochondrial Respiratory Chain
Mitochondrial cytopathies describe disorders for which mitochondrial dysfunction plays a key role in the pathogenesis of tissue injury. Mitochondria are the main site of intracellular energy production by the respiratory chain in all tissues, and the consequences of dysfunction are related to the energy needs of the affected tissue at that time. These can be protean in their manifestation including, but not limited to, myopathy, lactic acidosis, seizures, ataxia, peripheral neuropathy, blindness, deafness, gastrointestinal dysmotility, bone marrow dysfunction, liver failure, and chronic liver disease. Hepatic phenotypes may occur in isolation but more commonly are one component of a multisystemic disease.
Mitochondrial cytopathies are usually primary genetic disorders but may be secondary to a toxic insult. The overall incidence of mitochondrial cytopathies is one in 7500 live births, and approximately 20% will have liver involvement.
The underlying genetic disorder may be encoded in either mitochondrial (mtDNA) or nuclear DNA (nDNA). mtDNA is a separate 16.6 kb genome of maternal origin, which encodes two ribosomal RNAs, 22 transfer RNAs, and 13 subunits of the respiratory chain. nDNA contains approximately 1000 mitochondrial genes that encode most subunits of the respiratory chain and all of complex 2 subunits. In addition, they encode the proteins that control transcription, translation, salvage, and repair of mtDNA. Mutations in nDNA cause more than 80% of mitochondrial cytopathies and these are usually autosomal recessive. The most common entity is mtDNA depletion syndromes where mtDNA levels are low but qualitatively normal. Pathogenetic qualitative abnormalities in mtDNA include point mutations and either deletions or duplications. However, normal wild-type mtDNA and mutant mtDNA can occur in the same cell, which is known as heteroplasmy.
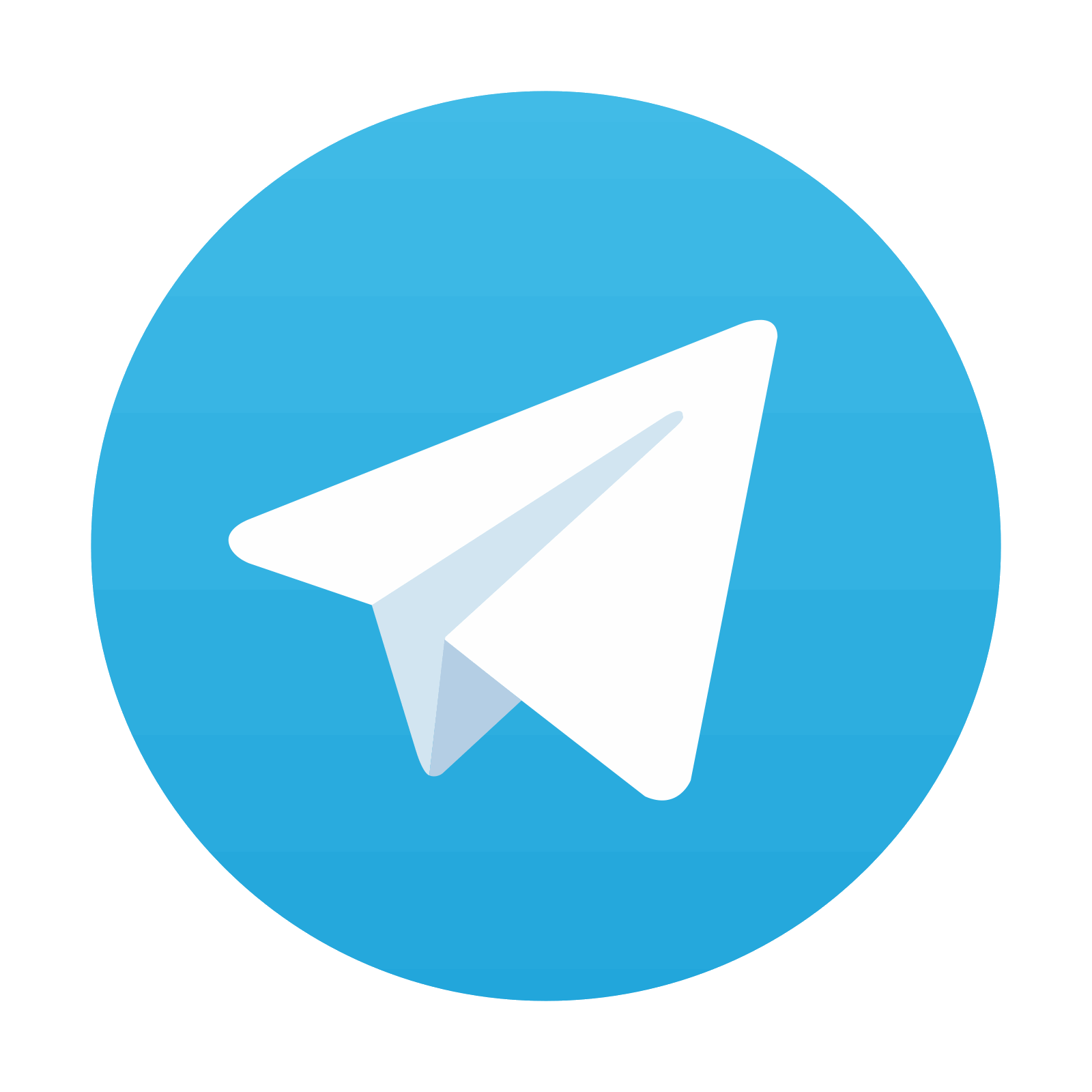
Stay updated, free articles. Join our Telegram channel
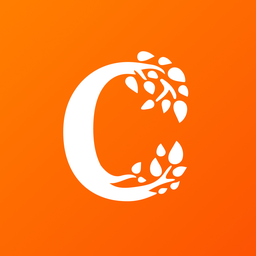
Full access? Get Clinical Tree
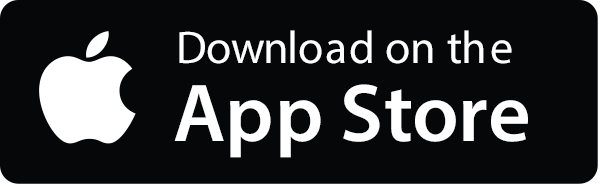
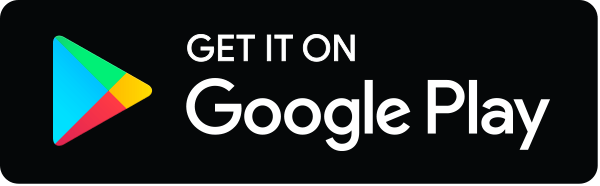