Abstract
Abnormalities in mineral metabolism occur early in the course of chronic kidney disease, even as early as stage 2, and progress as kidney function worsens. The progression of kidney disease is eventually associated with: (1) altered serum levels of calcium, phosphorus, parathyroid hormone, fibroblast growth factor 23, and vitamin D; (2) disturbances in bone modeling or remodeling with the development of fractures or impaired linear growth in children; and (3) extraskeletal calcification in soft tissues and arteries. Collectively, these abnormalities are called chronic kidney disease-mineral bone disorder (CKD-MBD).
Keywords
calcium, cardiovascular disease, CKD-MBD, fibroblast growth factor 23, parathyroid hormone, phosphorus, vascular calcification
Introduction
Chronic kidney disease (CKD) is associated with an increased risk of death and cardiovascular disease. Disorders of bone mineral metabolism and regulatory hormones seen in CKD are thought to play a key role in the excess morbidity and mortality. In people with healthy kidneys, normal serum levels of phosphorus and calcium are maintained through the interaction of three hormones: parathyroid hormone (PTH), 1,25(OH) 2 D (calcitriol, the active metabolite of vitamin D), and fibroblast growth factor 23 (FGF-23). These hormones act on three primary target organs: bone, kidney, and intestine. The kidneys play a critical role in the regulation of normal serum calcium and phosphorus concentrations, convert vitamin D into calcitriol, and respond to PTH and FGF-23. Thus derangements are common in patients with CKD. Abnormalities in mineral metabolism occur early in the course of CKD, even as early as stage 2, and progress as kidney function worsens. The progression of kidney disease is eventually associated with (1) altered serum levels of calcium, phosphorus, PTH, FGF-23 and vitamin D; (2) disturbances in bone modeling or remodeling with the development of fractures or impaired linear growth in children; and (3) extraskeletal calcification in soft tissues and arteries. Collectively, these abnormalities are called chronic kidney disease-mineral bone disorder (CKD-MBD) ( Box 10.1 ). In this chapter, the physiology and clinical consequences of CKD-MBD will be discussed, followed by treatment recommendations.
Definition of CKD-MBD
A systemic disorder of mineral and bone metabolism due to CKD manifested by either one or a combination of the following:
- •
Abnormalities of calcium, phosphorus, PTH, or vitamin D metabolism
- •
Abnormalities in bone turnover, mineralization, volume, linear growth, or strength
- •
Vascular or other soft tissue calcification
Definition of Renal Osteodystrophy
- •
An alteration of bone morphology in patients with CKD
- •
One measure of the skeletal component of the systemic disorder of CKD-MBD that is quantifiable by histomorphometry of bone biopsy
Phosphorus
Normal Physiology
Phosphorus is critical for numerous physiological functions, including skeletal development, mineral metabolism, cell membrane phospholipid content and function, cell signaling, platelet aggregation, and energy transfer through mitochondrial metabolism. Because of its importance, normal homeostasis maintains serum phosphorous concentrations between 2.5 and 4.5 mg/dL (0.81 to 1.45 mmol/L). The serum phosphorus concentration is maintained under the direction of hormonal regulators including FGF-23, PTH, and calcitriol. In an average diet, approximately 1 gram of phosphorus is ingested daily. Of this, 300 mg is excreted in the stool and 700 mg is absorbed. Eighty-five percent of phosphorus is contained in bone in the form of hydroxyapatite. Of the remainder, 14% is intracellular, and only 1% is extracellular. Of this extracellular phosphorus, 70% is organic (phosphate) and contained within phospholipids, and 30% is inorganic. The inorganic fraction is 15% protein bound, and the remaining 85% is either complexed with cations or circulates as the free monohydrogen or dihydrogen forms. Thus serum measurements only reflect a minor fraction of total body phosphorus and therefore do not accurately reflect total body stores in the setting of the abnormal homeostasis that occurs in CKD. The terms phosphorus and phosphate are often used interchangeably, but the term phosphorus means the sum of the two physiologically occurring inorganic ions in serum, hydrogen phosphate (HPO 4 2− ), and dihydrogen phosphate (H 2 PO 4 − ).
Most of the phosphorus (60% to 70%) is absorbed in the duodenum and jejunum with minimal absorption occurring in the ileum. Phosphorus absorption is dependent on both passive transport, related to the concentration in the intestinal lumen, and active transport stimulated by calcitriol, the active metabolite of vitamin D. The passive absorption is dependent on luminal phosphate concentration and occurs via the epithelial brush border sodium-phosphorus cotransporter (Npt2b). Calcitriol can upregulate the sodium-phosphate cotransporter and therefore actively increase phosphate absorption; however, there is near normal intestinal absorption of phosphorus in the absence of vitamin D. Medications or foods that bind intestinal phosphorus (antacids, phosphate binders, and calcium) can decrease the net amount of phosphorus absorbed by decreasing the free phosphate for absorption.
Most inorganic phosphate is freely filtered by the glomerulus. Approximately 70% to 80% of the filtered load is reabsorbed in the proximal tubule, which serves as the primary regulated site of the kidney. The remaining 20% to 30% is reabsorbed in the distal tubule. Factors that increase phosphorus excretion are primarily increased plasma phosphate concentration, PTH, and FGF-23. Conversely, acute or chronic phosphorus depletion will decrease excretion. Renal phosphorus excretion is also increased, although to a lesser extent, by volume expansion, metabolic acidosis, glucocorticoids, calcitonin, growth hormone, and thyroid hormone. The majority of this regulation occurs in the proximal tubule via sodium-phosphorus cotransporters. Similar to the intestine, the sodium-phosphorus cotransporters can be acutely moved to the brush border in the presence of acute or chronic phosphorus depletion. Alternatively, after a phosphorus load or in the presence of PTH, the exchanger is removed from the brush border and catabolized.
Renal phosphorus excretion is exquisitely sensitive to changes in the serum phosphorus level due to hormones that regulate phosphate excretion including PTH and FGF-23 (both are discussed in detail later). The normal homeostatic response to increased phosphorus levels is increased PTH and FGF-23. Both PTH and FGF-23 increase phosphorus excretion, but FGF-23 is the principle phosphaturic hormone. FGF-23 is predominately produced from bone cells (osteocytes and bone-lining cells) during active bone remodeling, but messenger RNA is also found in heart, liver, thyroid/parathyroid, intestine, and skeletal muscle. FGF-23 requires the coreceptor klotho for binding to its receptor as inactivating klotho in FGF-23 overexpressing mice reverses biochemical and skeletal abnormalities. Klotho is found in the distal tubule and is downregulated in aging and CKD. Once active, FGF-23 regulates Npt2a independently of PTH and affects the conversion of 25 hydroxyvitamin D to calcitriol (1,25[OH] 2 D) by inhibition of the 1α-hydroxylase enzyme in the renal tubules, leading to hypophosphatemia and inappropriately normal or low calcitriol levels. FGF-23 also stimulates PTH. Mice with targeted ablation of FGF-23 confirm these physiological effects of FGF-23: hyperphosphatemia, inappropriately low PTH, increased calcitriol, and bone loss. Overexpression of FGF-23 in mice leads to the progressive development of secondary hyperparathyroidism. FGF-23 gene expression in bone is regulated by both phosphorus and calcitriol, even in uremic animals. In contrast, PTH stimulates 1α-hydroxylase activity, thereby increasing the production of 1,25(OH) 2 D, which in turn negatively feeds back on the parathyroid gland to decrease PTH secretion. Because PTH is also stimulated in response to hypocalcemia, this proposed homeostatic loop implies that the effects of PTH would predominate in the setting of high phosphorus and low calcium, whereas FGF-23 would predominate in the setting of high phosphorus and normal or high calcium.
Abnormal Physiology in Chronic Kidney Disease
The ability of the kidneys to control phosphate becomes impaired at glomerular filtration rates (GFRs) of approximately 50 to 60 mL/min/1.73 m 2 . Frank hyperphosphatemia is not observed in most subjects until the GFR is less than 25 to 30 mL/min/1.73 m 2 . Although phosphorus levels are maintained in the “normal” range in patients with CKD stages 3 and 4 (GFR 30 to 60 mL/min/1.73 m 2 and 15 to 29 mL/min/1.73 m 2 , respectively), there is a gradual increase in the serum level with progressive CKD. Phosphate retention plays a pivotal role in the development of CKD-MBD ( Fig. 10.1 ). With the initial fall in GFR there is a decrease in the amount of filtered phosphate and subsequently excreted phosphate. The remaining nephrons increase single-nephron phosphate excretion due to rises in FGF-23 and PTH. FGF-23 rises very early in CKD, before changes in the serum calcium phosphorus or PTH levels. The rise in FGF-23 leads to a decrease in calcitriol levels inhibiting intestinal phosphate absorption but also decreasing serum calcium levels and subsequently leads to a rise in serum PTH ( Fig. 10.2 ). The rise in serum PTH and FGF-23 at the expense of maintaining normal serum phosphorus is often referred to as the trade off hypothesis . Phosphorus loading increases FGF-23 and PTH, and conversely, phosphorus restriction inhibits the rise in PTH. Additional studies in isolated parathyroid glands or cells confirm a direct role of phosphorus on the regulation of PTH synthesis through multiple mechanisms. Hyperphosphatemia also indirectly increases PTH by inhibiting the activity of 1α-hydroxylase, thereby reducing the conversion of 25(OH)D to 1,25(OH) 2 D. In the skeleton, phosphorus stimulates FGF-23 secretion from osteocytes.


Clinical Consequences of Abnormal Phosphate
Higher serum phosphate levels consistently associate with clinical and subclinical cardiovascular disease among patients with and without kidney disease. Adverse effects occur both from consequences of hyperphosphatemia and elevations in hormones designed to maintain phosphate balance. Hyperphosphatemia is associated with increased vascular stiffening, arterial calcification, calciphylaxis, and valvular calcification. Epidemiological data suggest that serum phosphorus levels above the normal range are associated with increased morbidity and mortality in patients with CKD. These studies differ in their sample size, analyses, and their chosen reference range. The inflection point or range at which phosphorus becomes significantly associated with increased all-cause mortality in dialysis patients varies between studies being 5.0 to 5.5 mg/dL (1.6 to 1.8 mmol/L), greater than 5.5 mg/dL (>1.8 mmol/L), 6.0 to 7.0 mg/dL (1.9 to 2.3 mmol/L), and greater than 6.5 mg/dL (>2.1 mmol/L). A Dialysis Outcomes and Practice Patterns Study (DOPPS) analysis demonstrates that the relationship between elevations in serum phosphorus and mortality is consistent across all countries analyzed and that if a facility had 10% more patients with phosphorus levels between 6.1 to 7.0 mg/dL and greater than 7.0 mg/dL (1.97 to 2.26 mmol/L and greater than 2.26 mmol/L), mortality risk was 5.3% and 4.3% higher, respectively. Even in the nondialysis population, higher levels of serum phosphorus, even within the normal range, have been associated with increased risk of all-cause or cardiovascular mortality in patients with normal renal function who were free of cardiovascular disease, in patients with coronary artery disease and normal renal function, and in patients with CKD stages 3 through 5. In addition, hyperphosphatemia is a risk factor for more rapid progression of CKD. Increases in FGF-23 resulting from disordered phosphate metabolism may be a greater risk factor for cardiovascular disease and death than serum phosphorus. Higher FGF-23 levels are associated with increased mortality, cardiovascular disease, left ventricular hypertrophy, vascular calcification, and progression of kidney disease. Thus there is clear epidemiological data to support that patients with lower levels of phosphorus do better. Unfortunately, no study has demonstrated that lowering the serum phosphorus to a specific value leads to improved outcomes.
Management of Elevated Phosphate in Chronic Kidney Disease
Normalizing serum phosphorus is an important therapeutic goal for CKD-MBD. Unfortunately, it remains challenging and requires a combination of dietary restriction, phosphate binders, and enhanced dialytic removal. It should be noted that treatment of CKD-MBD should be based on serial assessments of phosphate, calcium, and PTH levels. There is great interdependency of these parameters and therapies aimed at only one often has unintentional effects on another parameter. Thus phosphate, calcium, and PTH levels should be considered together when making management decisions. The Kidney Disease: Improving Global Outcomes (KDIGO) guidelines recommend focusing treatment on patients with hyperphosphatemia.
Diet
Phosphorus is contained in almost all foods. Unfortunately, foods high in phosphate are generally also high in protein, making it challenging to balance dietary phosphorus restriction against the need for adequate protein intake in patients with CKD. Indeed, most well-nourished dialysis patients are in positive phosphorus balance. Roughly 60% to 70% of consumed phosphorus is absorbed, so about 4000 to 5000 mg of phosphate per week enters the extracellular fluid. Phosphate is often added to processed foods, and the amount of phosphate from these sources is significant but difficult to quantify as manufacturers are not required to list the amount of phosphate in foods. Prescription medications, over the counter medications, and dietary supplements have been found to be hidden sources of phosphate. Data regarding the amount of phosphate contained in the medications is limited but data does suggest that they contribute significantly to the phosphate load due to the large number of medications that CKD patients take. The KDIGO 2017 guidelines recommend limiting dietary phosphate intake in patients with CKD stages 3 to 5D. The source of phosphorus (organic vs. inorganic) also needs to be taken into consideration as the bioavailability of phosphorus is different. Organic phosphate is found in animal- and plant-based foods whereas phosphate additives contain inorganic phosphate. Forty percent to 80% of animal-based phosphate and 20% to 50% of plant-based phosphate is absorbed, whereas greater than 90% of phosphate in additives is absorbed. Educating patients on how to read food labels is helpful in reducing phosphate intake. Nutrition education should focus on how to help patients make the best choices as restricting phosphate must not compromise adequate protein intake. Dietary phosphate restriction alone, although an important component of effective phosphorus management, is usually not sufficient to control serum phosphate levels in most dialysis patients.
Phosphate binders
Phosphate binders are the mainstay of treatment of hyperphosphatemia in dialysis patients. Nearly all dialysis patients are prescribed binders. In the nondialysis population there is lack of data demonstrating efficacy of phosphate binders for lowering serum phosphate. A recent trial comparing placebo to active phosphate binder therapy in patients with CKD stages 3B to 4 found a minimal decline in serum phosphate from 4.2 mg/dL at baseline to 3.9 mg/dL after 9 months. Active binder therapy also resulted in a significant increase in coronary artery and abdominal aorta calcification. Although the data suggest that this observed increase in calcification was due to calcium-based binders, those treated with noncalcium-based binders had no advantage over placebo in regard to calcification. Thus the updated recommendations by KDIGO are to focus treatment on CKD stage 3 to 4 patients with hyperphosphatemia, and decisions about treatment should be based on progressive or persistently elevated serum phosphate.
The current phosphate binders available work by binding phosphate in the gastrointestinal (GI) tract and excreting phosphate in feces. Current binders are based on metals (aluminum, lanthanum), calcium, magnesium, polymers, and iron. Advantages and disadvantages of the currently available binders are shown in Table 10.1 .
Binder | Advantages | Disadvantages | Forms | Dosage (mg) |
---|---|---|---|---|
Calcium Carbonate |
|
|
|
|
Calcium Acetate |
|
|
|
|
Magnesium Carbonate/Calcium Acetate |
|
|
|
|
Aluminum hydroxide |
|
|
|
|
Lanthanum Carbonate |
|
|
|
|
Sevelamer hydrochloride |
|
|
|
|
Sevelamer Carbonate |
|
|
|
|
Sucroferric Oxyhydroxide |
|
|
|
|
Ferric Citrate |
|
|
|
|
The use of aluminum as a phosphorus binder was popular throughout the 1970s and 1980s. But as evidence emerged implicating aluminum’s role in osteomalacia and dialysis encephalopathy, the therapeutic trend shifted to the use of calcium carbonate. In the decade that followed, it was noted that calcium acetate would reduce the elemental load of calcium while controlling the serum phosphorus level compared with calcium carbonate, although there was no difference in the incidence of hypercalcemia. Subsequent studies demonstrated that the calcium-containing phosphate binders were associated with increased burden of vascular calcification in some, but not all studies. This and the increased risk of hypercalcemia with the concomitant use of vitamin D led to a need for noncalcium-containing, nonaluminum-containing phosphate binders. In 1998 sevelamer hydrochloride (HCl) was introduced and was shown to be as effective as calcium acetate in maintaining the serum phosphorus without hypercalcemia in crossover trials.
It is now recommended to restrict the dose of calcium-based phosphate binders in adult patients with CKD due to data suggesting that excess exposure to calcium (either through diet or medications) may be harmful in patients with CKD regardless of GFR. Two recent randomized controlled trials (RCTs) in predialysis and dialysis patients found a significant survival benefit in patients treated with sevelamer versus calcium-containing binders over a 3-year period. Dialysis patients treated with sevelamer had a 10-fold reduction in the risk of experiencing a lethal cardiac arrhythmia and all-cause cardiovascular death compared with patients treated with calcium-based binders. However, one of the largest studies to date, the Dialysis Clinical Outcomes Revisited (DCOR) trial, in which 2103 dialysis patients were randomized to either sevelamer or a calcium-based binder did not find a difference in all-cause or cause-specific mortality rates between the two groups. The study did find a decrease in all-cause hospitalizations with sevelamer. The very high dropout rate (47% in the sevelamer arm and 51% in the calcium-based arm) made the study underpowered; thus it should not be considered a negative study, but rather an inadequate study.
Studies have also found that calcium-based binders may worsen vascular calcification. In a nonblinded study called the Treat-to-Goal study, patients were randomized to either a calcium-based binder or sevelamer HCl for 1 year. Despite equivalent phosphorus control, patients taking a calcium binder had progression in coronary and aorta calcification, whereas patients treated with sevelamer HCl did not progress. In an open-label extension of this study in Europe for a second year, calcification scores continued to rise significantly in patients treated with a calcium-based binder, but not in the sevelamer HCl group. The Renagel in New Dialysis Patients (RIND) study found that approximately 65% of patients new to dialysis have coronary artery calcification. In this 18-month trial, 60 incident hemodialysis patients were randomized to calcium-based binders and 54 to sevelamer HCl. Similar to the Treat-to-Goal study, patients had equivalent control of serum phosphorus levels, yet patients treated with calcium-based binders had progressive calcification and those treated with sevelamer HCl did not. In a study of 90 binder-naive patients with CKD stages 3 to 5 who were not receiving dialysis, Russo and colleagues randomized patients (30 per group) to either a low-phosphate diet alone, a low-phosphate diet in combination with fixed doses of calcium carbonate (2 g/day), or a low-phosphate diet in combination with sevelamer HCl (1600 mg/day), and they followed these individuals for 2 years. The primary endpoint of the study was progression of coronary artery calcification, assessed as the total calcium score using multislice spiral computed tomography (CT). Among the 84 patients who completed the study, final coronary artery calcification scores were greater than initial scores in those receiving diet alone ( P < 0.001) or diet in combination with calcium carbonate ( P < 0.001), whereas there was no progression of calcification in the group treated with diet plus sevelamer HCl. Thus three studies found less calcification with the use of sevelamer HCl compared with calcium-based binders. Two studies have failed to find differences in coronary artery calcification with sevelamer HCl compared with calcium-based binders. In the CARE 2 study, chronic hemodialysis patients from the United States were randomized to receive either calcium acetate or sevelamer HCl. The study was designed to assess noninferiority, evaluating coronary artery calcification by electron beam computed tomography (EBCT) at 6 and 12 months after randomization. There was no difference in the progression of arterial calcification between groups. It should be noted though that before 1 year, 30% of patients in the sevelamer HCl arm and 43% in the calcium acetate arm dropped out, leaving the final sample size less than the power needed to determine noninferiority. The second study was the Brazilian Renagel and Calcium (BRIC) study that compared calcium acetate versus sevelamer on coronary artery calcification and bone histomorphometry in hemodialysis patients. The annual rates of progression of coronary calcification scores were not statistically different. However, this study was hampered by several significant confounders: differences in baseline coronary artery calcification scores between the two study arms; the use of high dialysate calcium concentrations in most patients; and multiple interventions during the course of the study allowed by the caring physicians based on bone biopsy results.
Another noncalcium binder, lanthanum carbonate, was introduced in 2005. Lanthanum carbonate effectively binds intestinal phosphorus without sequestering bile acids and is poorly absorbed. The tablets are chewable and well tolerated. Randomized prospective studies have demonstrated that lanthanum carbonate controls serum phosphorus as well as other binders, but with less hypercalcemia. Although there has been concern of toxicities similar to aluminum, the clearance of lanthanum is primarily by the liver as opposed to renal clearance for aluminum. Although animal studies are controversial, in human studies no liver toxicity, suppression of erythropoiesis, or change in the mental status examination have been observed. In addition, no direct bone toxicity has been demonstrated. In a randomized, open-label, multicenter study where bone biopsies were performed, lanthanum did not induce osteomalacia, whereas treatment with calcium carbonate increased the incidence of adynamic bone disease. To date, there are no human studies demonstrating that lanthanum carbonate can prevent vascular calcification.
There are now two available iron-based phosphate binders in the United States: sucroferric oxyhydroxide and ferric citrate. An open-label, phase III, randomized, active-controlled, parallel-group, multicenter study was performed in 1059 dialysis patients ( n = 968 hemodialysis patients, n = 86 peritoneal dialysis patients) over 24 weeks examining sucroferric oxyhydroxide compared with sevelamer. The study demonstrated that sucroferric oxyhydroxide was noninferior to sevelamer for control of serum phosphate. In addition, treatment effect was achieved with 62% fewer sucroferric oxyhydroxide tablets than sevelamer. Transferrin saturation increased significantly in the sucroferric oxyhydroxide group. There was a trend toward increased ferritin levels in the sucroferric oxyhydroxide group but it did not reach statistical significance. Adverse events were more common in the sucroferric oxyhydroxide group than the sevelamer group (83.2% vs. 76.1%). The most frequent adverse events with sucroferric oxyhydroxide were diarrhea, discolored stools, and hyperphosphatemia. The long-term effects of sucroferric oxyhydroxide were examined in a 28-week extension study of the phase III study. Six hundred and forty-four patients participated in the extension study and were continued on the same treatment and dose of phosphate binder that they were receiving at the end of the initial study. Serum phosphate remained within the Kidney Disease Outcomes Quality Initiative (KDOQI) target range for both treatment groups throughout the study. The number of daily tablets was lower in the sucroferric oxyhydroxide group (mean ± SD, 4.0 ± 1.5 tablets/day) compared with sevelamer (mean ± SD, 10.1 ± 6.6 tablets/day). There was no evidence of iron accumulation over the 1-year period of treatment. There were no significant changes in transferrin saturation, ferritin, iron, or hemoglobin concentrations between the two groups. Adverse events were more common with sucroferric oxyhydroxide compared with sevelamer (14.6% vs. 9.0%) and the most common events reported were hypophosphatemia and hyperphosphatemia. Overall, sucroferric oxyhydroxide appears to be an effective and well-tolerated phosphate binder. One disadvantage is that it inhibits GI absorption of levothyroxine, so it cannot be prescribed with levothyroxine.
Ferric citrate is another iron-based binder approved for use in dialysis patients in the United States. In a large, phase III study of >400 dialysis patients randomized to ferric citrate or active control for 56 weeks, ferric citrate was as effective as sevelamer and calcium acetate in controlling serum phosphate levels. Ferric citrate had a lower average pill burden compared with sevelamer (8 tablets/day vs. 9 tablets/day, P = 0.01) but did not have lower pill burden than calcium acetate (8 tablets/day vs. 7.7 tablets/day, P = 0.28). More patients in the ferric citrate group discontinued the study drug compared with the active control in the first weeks of the study (21% vs. 15%). The most common reason for discontinuation of the study drug was nonserious GI side effects. Thirty-nine percent of patients in the ferric citrate group experienced a serious adverse event compared with 49% in the active control group. There was a significant increase in serum ferritin and transferrin saturation in the ferric citrate group. In addition, ferric citrate reduced both intravenous iron and erythropoietin stimulation agent (ESA) usage at 52 weeks. Hemoglobin levels remained stable throughout the study period and were slightly higher in the ferric citrate group. There was no evidence of iron accumulation throughout the study period but the study did not perform radionuclide-tagged balance studies to confirm.
Overall, the iron-based binders appear to be effective with minimal side effects and less pill burden than calcium-based or polymer-based binders. The long-term effects of these medications beyond 1 year have not been determined and there are no studies examining patient outcomes with these binders.
A new agent for the management of hyperphosphatemia has been developed. Tenapanor is a small molecule inhibitor of the sodium/hydrogen exchanger isoform 3 that specifically targets the transepithelial transport of intestinal phosphate. It reduces the absorption of sodium and phosphate in the gut. In a randomized trial of 162 hemodialysis patients randomized to tenapanor versus placebo, tenapanor resulted in a dose-dependent reduction in serum phosphate from baseline (least-squares mean change: tenapanor = 0.47 to 1.98 mg/dL; placebo = 0.54 mg/dL; P = 0.01). Diarrhea was the most common adverse event. A phase III trial is currently underway in dialysis patients. Tenapanor is not currently approved for the treatment of hyperphosphatemia in the United States.
The KDIGO guidelines for CKD-MBD now recommend restricting the dose of calcium-based phosphate binders in patients with CKD regardless of whether other markers of risk such as arterial calcification, hypercalcemia, low PTH levels, or adynamic bone disease are also present. It should be noted that none of the studies provide dose-threshold information nor do they give information on moderately dosed calcium-based binders in combination therapies, so there is no current guideline about a maximum dose. In clinical practice, economic factors, patient preference, and GI distress limit adherence to a rigorous diet and binder schedule. The choice of binder should be individualized for each patient because in the end, the best phosphate binder is what works for an individual patient. Ultimately, there are no studies that demonstrate that lowering the phosphorus to a specific level improves mortality or reduces cardiovascular risk in patients with CKD.
Dialysis
Dialysis is an important part of phosphate management in patients on dialysis. Conventional dialysis removes 1000 mg of phosphorus per dialysis, but because 1000 mg is absorbed on a daily basis, the net positive balance may be 4000 mg per week. Patients who undergo nightly dialysis have weekly removal of phosphate that is twice as high. In a study of 10 patients dialyzing 8 to 10 hours, 6 to 7 days per week at blood flows of 100 mL/min/1.73 m 2 , it became possible to discontinue binder therapy and increase dietary phosphate (and protein) intake. One prospective RCT has reported the impact of alternative dialysis therapies using biochemical markers of CKD-MBD as a secondary endpoint. In this study, 26 patients receiving nocturnal, prolonged-duration, hemodialysis six times weekly were compared with 25 patients receiving standard hemodialysis given three times weekly for 4 hours each session in a parallel design. The authors found significant decreases in serum phosphorus and intact PTH, but no difference in calcium in the patients allocated to frequent nocturnal hemodialysis. Importantly, the phosphate-binder dose was also reduced. These data suggest that frequent nocturnal hemodialysis can lead to an improvement in mineral metabolism. Thus optimized control of serum phosphorus may require the use of alternative dialytic regimens in addition to diet and phosphate binders in some patients.
Calcium
Normal Physiology
Maintenance of calcium levels within a narrow physiological range is essential to life. Calcium homeostasis is complex and tightly regulated and depends on regulation of calcium fluxes within the intestine, kidney, and bones. The serum calcium level is a poor reflection of overall total body calcium, as serum levels are less than 1% of total body calcium; the remainder is stored in bone. Ionized calcium, generally 40% of total serum calcium levels, is physiologically active, whereas the nonionized calcium is bound to albumin or anions such as citrate, bicarbonate, and phosphate. In the presence of hypoalbuminemia, there is an increase in the ionized calcium relative to the total calcium, thus total serum calcium may underestimate the physiologically active (ionized) serum calcium. A commonly used formula for estimating the ionized calcium from total calcium is to add 0.8 mg/dL for every 1 mg decrease in serum albumin less than 4 mg/dL. However, data in CKD patients has demonstrated that this formula offers no superiority over total calcium alone and is less specific than ionized calcium measurements. In addition, the assay used for albumin may affect the corrected calcium measurement. Thus ionized calcium should be measured if more precise assessment of serum calcium levels is needed.
GI absorption of calcium is a highly selective process as only 20% to 25% of total dietary calcium is absorbed. Calcium absorption across the intestinal epithelium occurs via a vitamin D–dependent, saturable (transcellular) TRPV5 and TRPV6 transporters (animal homologues ECaC2 and CaT1) and independent, nonsaturable (paracellular) pathways. The intracellular calcium then associates with calbindin 9k to be “ferried” to the basolateral membrane where calcium is removed from the enterocytes via the calcium-ATPase. TRPV6 is the main transporter responsible for intestinal calcitriol-dependent calcium absorption, with compensation by TRPV5 when needed. Many factors regulate intestinal calcium absorption. Age, vitamin D and calcium intake, serum levels of calcitriol, and PTH all regulate calcium absorption. Calcitriol is the most important hormonal regulatory factor because it induces calcium channels, calbindin (which binds calcium and removes calcium from the microvilli region), and increases the Ca 2+ -ATPase.
The kidneys play a key role in calcium regulation. Ninety-eight percent to 99% of the filtered load of calcium is reabsorbed by the renal tubules. The majority (60% to 70%) of calcium is reabsorbed passively in the proximal tubule driven by a transepithelial electrochemical gradient that is generated by sodium and water reabsorption. In the thick ascending limb, another 20% of calcium is reabsorbed via paracellular transport. Only 5% to 10% of the filtered calcium load is reabsorbed in the distal nephron, but the distal nephron is the major site for regulation of calcium excretion. Although the majority of total renal calcium reabsorption is paracellular, the regulation of reabsorption is via transcellular pathways that occur in the distal convoluted tubule, the connecting tubule, and the initial portion of the cortical collecting duct. The calcium enters these cells via TRPV5 and TRPV6 calcium channels down electrochemical gradients, binds with calbindin 28k, and is transported to the basolateral membrane where calcium is actively reabsorbed by the Na 2+ /Ca 2+ exchanger (NCX1) and/or the Ca 2+ -ATPase (PMCA1b). Both TRPV5 and TRPV6 are localized to these distal nephron segments, with upregulation by calcium, PTH, vitamin D, and estrogen. TRPV5 is the most critical, with compensation by TRPV6, which is the opposite from compensation in the intestine.
PTH is the most important regulator of serum calcium. Small reductions in serum ionized calcium lead to the rapid release of PTH due to the existence of a calcium sensor in the parathyroid glands. The calcium-sensing receptor (CaR) belongs to a super family of G-protein–coupled receptors. Activation of the CaR stimulates phospholipase C, leading to increased inositol increased inositol 1,4,5-triphosphate (IP3), which mobilizes intracellular calcium and decreases PTH secretion. In contrast, inactivation reduces intracellular calcium and increases PTH secretion. The CaR is expressed in organs controlling calcium homeostasis, such as parathyroid gland, thyroid C cells, intestine, kidney, and likely bone. In the kidney, the CaR is expressed in mesangial cells and throughout the tubules. The CaR is found not only on the apical membrane of the proximal tubule and the inner medullary collecting duct but also on the basolateral membrane of the medullary and cortical thick ascending limb and distal convoluted tubule. Activation of CaR on the thick ascending limb leads to increased intracellular free Ca 2+ , which decreases paracellular calcium reabsorption. This CaR also responds to increases in intraluminal calcium concentration by reducing antidiuretic hormone-stimulated water absorption. In theory, this may provide a mechanism by which the urine can stay dilute in the face of hypercalcemia and hypercalciuria to avoid dangerous calcium precipitation, and it may explain the polyuria observed in patients with hypercalciuria. The expression of the CaR is regulated by calcitriol in parathyroid, thyroid, and kidney cells, but the renal effects of CaR are both dependent and independent of PTH. In uremic animals, the expression of CaR in the parathyroid gland is downregulated by high phosphorus diet and occurs after the onset of parathyroid hyperplasia. Once downregulated, the expression can be rescued by a low phosphorus diet.
Calcitriol is another key factor controlling calcium absorption. Cholesterol is synthesized to 7-dehydrocholesterol, which in turn is metabolized in the skin to vitamin D 3 . In addition, there are dietary sources of vitamin D 2 (ergocalciferol) and vitamin D 3 (cholecalciferol). Once in the blood, vitamin D 2 and D 3 bind with vitamin D–binding protein (DBP) and are carried to the liver where they are hydroxylated by CYP27A1 in an essentially unregulated manner to yield 25(OH)D, often called calcidiol and measured as “vitamin D.” Calcidiol is then converted in the kidney to calcitriol by the action of 1α-hydroxylase ( CYP27B1 ). The 1α-hydroxylase enzyme in the kidney is also the site of regulation of calcitriol synthesis by numerous other factors, including low calcium, low phosphorus, estrogen, prolactin, growth hormone, calcitriol itself, and FGF-23. Calcitriol mediates its cellular function via both nongenomic and genomic mechanisms. Calcitriol facilitates the uptake of calcium in intestinal and renal epithelium by increasing the activity of the voltage-dependent calcium channels TRPV5 and TRPV6, upregulating the calcium transport protein calbindins and the basolateral calcium-ATPase. It also is essential for normal bone remodeling.
Abnormal Physiology in CKD
Normal calcium homeostasis is interrupted early in the course of CKD. An increase in FGF-23 levels is the earliest abnormality. Rising FGF-23 levels inhibit production of calcitriol through inhibition of 1α-hydroxylase resulting in decreased calcium reabsorption. In response, PTH levels increase to maintain serum calcium levels. Thus serum calcium levels are maintained in the normal range, at the expense of hyperparathyroidism, throughout the course of CKD until the GFR is less than 30 mL/min/1.73 m 2 . Late in the course of CKD, calcitriol levels are inadequate to increase intestinal calcium absorption. In addition, most patients with CKD stages 3 and 4 have very low levels or urinary calcium excretion, suggesting maximum tubular reabsorption.
The inability to maintain normal calcium homeostasis in CKD may lead to inappropriate calcium balance. In CKD, the bone appears less able to take up calcium, at least in low-turnover states that can be present in up to 50% of patients with CKD. This has led to the concept of “calcium loading” when patients are also given a calcium-based binder, because, even with normal serum levels, excess calcium intake can lead to a positive calcium balance. A study of eight patients with CKD (mean estimated GFR [eGFR] 36 mL/min/1.73 m 2 ) found that patients were in neutral calcium balance while consuming a 957 mg calcium diet, but they went into markedly positive calcium balance of 500 mg per day when treated with calcium carbonate. These data support findings from another study in patients with CKD stages 3 and 4 showing positive calcium balance while consuming a diet of 2000 mg/day calcium. Without net urinary excretion or bone uptake, a positive calcium balance may predispose the person to extraskeletal calcification. In CKD patients, approximately 18% to 20% of calcium is absorbed from the intestine. If patients are taking 2000 mg/day in total elemental calcium intake (1500 mg from binder and 500 mg from diet), and 20% is absorbed, then the net intake is 400 mg per day. On hemodialysis days, this figure is slightly greater because approximately 50 mg of calcium is infused with a 4-hour dialysis treatment using 2.5 mEq/L dialysate calcium concentration. In patients on peritoneal dialysis, there is a slight efflux of calcium using a 2.5 mEq/L dialysate in four daily exchanges. Thus the absorbed intake of elemental calcium from a 2000 mg elemental calcium diet (plus binders) and 2.5 mEq/L calcium dialysate would be 350 to 450 mg/day. In patients taking most forms of vitamin D, net intestinal absorption would be enhanced, thereby increasing the net intake further. In an anuric patient, this positive balance of calcium load has only two “compartments” to go to: bone and extraskeletal locations. If the bone is normally remodeling, then the calcium should be deposited there; however, normal bone is not common in dialysis patients (discussed later). If no calcium-containing phosphate binder is taken, then the patients should be in a neutral or slightly negative balance depending on stool and sweat output. The fraction of the excess calcium that is deposited into bone or into soft tissues (which could promote calcification) is difficult to determine. The KDIGO 2017 guidelines recommend restricting the dose of calcium-based phosphate binders in patients with CKD regardless of whether other markers of risk such as arterial calcification, hypercalcemia, low PTH levels, or adynamic bone disease are also present.
Clinical Consequences of Abnormal Calcium Homeostasis
Both hypocalcemia and hypercalcemia are associated with increased mortality in patients with CKD. Higher serum calcium levels are associated with increased mortality and cardiovascular events in patients with CKD. Hypercalcemia can also lead to arrhythmias. As discussed previously, positive calcium balance may lead to vascular calcification, which is associated with an increased risk of cardiovascular disease and mortality in CKD patients. Hypocalcemia contributes to secondary hyperparathyroidism (SHPT) and renal osteodystrophy. High-turnover bone disease is observed in patients with SHPT. In addition, SHPT is associated with an increased risk for cardiovascular events and death. It is unclear at what level of low serum calcium there is an increased risk. It is also important to realize that none of these studies evaluated patients receiving cinacalcet, which lowers calcium by its effects on the CaR, with an expected decrease in the total serum calcium concentration. Thus we do not know whether patients with low serum calcium levels due to cinacalcet have a similar risk to those with similar serum calcium levels who are not on the drug.
Management of Calcium in Chronic Kidney Disease
The KDIGO guidelines do not suggest limits for dietary calcium intake, but they do recommend restricting the dose of calcium-based binders for all patients with CKD stages 3 to 5D. The balance studies discussed previously are consistent in showing that 2000 mg/day of calcium intake is too high for patients with CKD stages 3 to 5D. A more moderate target for dietary calcium intake of 800 to 1000 mg/day may be more appropriate in patients with CKD. The updated KDIGO guidelines do not recommend correction of hypocalcemia in all patients. An individualized approach to the treatment of hypocalcemia should be used because those patients with symptomatic or severe hypocalcemia may benefit from correction. The choice of whether or not to supplement with calcium may also depend on the dietary calcium intake of the individual patient. For those patients with high calcium intakes (>1000 mg/day), calcium supplementation should probably be avoided.
The use of calcium-based phosphate binders also should be restricted in patients with CKD stages 3 to 5D. Several RCTs have shown significant survival benefits for patients treated with sevelamer versus calcium-containing binders. It is important to note that none of the available studies provide dose-threshold information about calcium exposure, so a maximum dose of calcium-based binders is unknown. Further research is needed to determine the optimal calcium intake for patients with CKD.
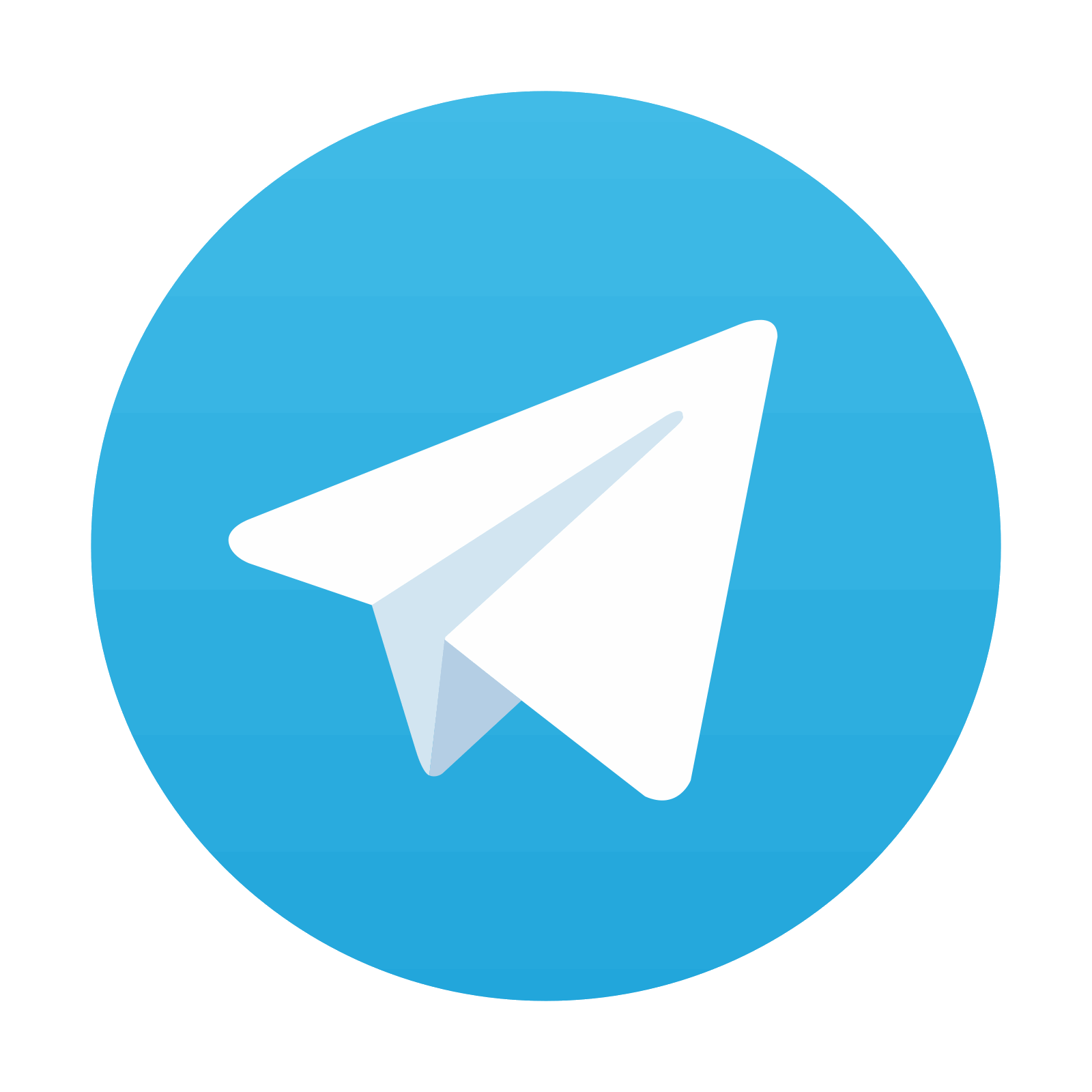
Stay updated, free articles. Join our Telegram channel
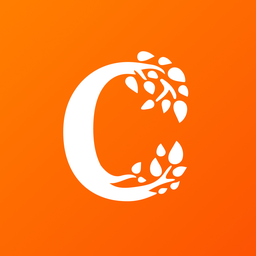
Full access? Get Clinical Tree
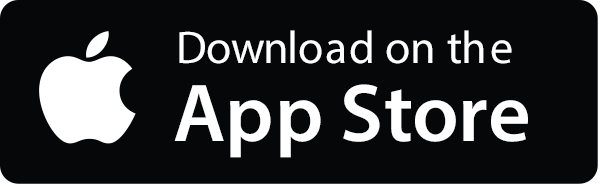
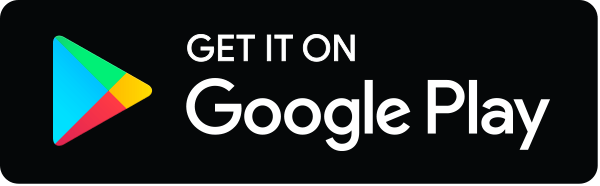