PATHOPHYSIOLOGY OF METABOLIC ALKALOSIS
Metabolic alkalosis is an acid–base disorder that occurs as the result of a process that increases pH (alkalemia) from a primary increase in serum bicarbonate concentration (). The primary elevation of serum [
] is caused by the pathophysiologic processes outlined below.
Net H+ Loss from Extracellular Fluid
A loss of protons from the body occurs primarily through either the kidneys or the gastrointestinal (GI) tract. When H+ losses exceed the daily H+ load produced by metabolism and diet, a net negative H+ balance results. Because the H+ loss results in the generation of a , increases in serum [
] result. GI loss of protons generally occurs in the stomach; in this setting, H+ secretion by the luminal gastric parietal cell H+-adenosine triphosphatase (ATPase) leaves a
to be reclaimed at the basolateral surface. The coupling between net acid excretion (NAE) and bicarbonate generation in the kidney was discussed at length in Chapter 7. Finally, H+ shifts into cells may accompany significant potassium depletion. Again, this should produce a rise in extracellular fluid (ECF) [
]. Regarding this last mechanism, we should point out that evidence of intracellular acidosis developing during experimental potassium depletion has not been consistently observed in experimental settings, and it is certainly possible that increases in NAE serves as the predominant mechanism for metabolic alkalosis with potassium deficiency.
Net Bicarbonate or Bicarbonate Precursor Addition to Extracellular Fluid
administration or addition of substances whose metabolism generates
(eg, lactate, citrate) at a rate greater than that of metabolic H+ production also leads to an increase in ECF [
]. In the presence of normal kidney function, however, ECF [
] will not increase significantly. This occurs because as serum [
] exceeds the plasma threshold for
reabsorption, the kidney excretes the excess
. As a result serum bicarbonate will not rise unless there is a change in renal bicarbonate handling (maintenance factor). The need for maintenance factors in the pathogenesis of metabolic alkalosis is discussed in more detail below.
Loss of Fluid from the Body That Contains Chloride in Greater Concentration and Bicarbonate in Lower Concentration Than Serum
If this type of fluid is lost, ECF volume must contract. If this contraction is substantial enough, a measurable increase in serum [] develops. Protons are not lost in this setting in contrast to losses noted with vomiting or nasogastric suction. Bicarbonate is now distributed in a smaller volume, resulting in an absolute increase in ECF [
]. This is referred to as contraction alkalosis.
KEY POINTS
COMPENSATORY MECHANISMS FOR METABOLIC ALKALOSIS
The first line of pH defense during metabolic alkalosis is, again, buffering. When is added to ECF, protons react with some of this
to produce CO2 that is normally exhaled by the lungs. Through this chemical reaction, the increase in serum and ECF [
] is attenuated. It has been shown that the intracellular fluid (ICF) contributes the majority of H+ used in this buffering process.
Respiratory compensation also occurs with metabolic alkalosis. Under normal conditions, control of ventilation occurs in the brainstem and is most sensitive to interstitial H+ concentration (see Chapter 9). Respiratory compensation to metabolic alkalosis follows the same principles as respiratory compensation to metabolic acidosis. Of course, the direction of the change of partial pressure of arterial carbon dioxide (PaCO2) is different (ie, hypercapnia as a result of hypoventilation rather than hypocapnia as a result of hyperventilation occurs) and constraints regarding oxygenation must limit the magnitude of this hypoventilatory response. With metabolic alkalosis, the PaCO2 should increase 0.6 to 1.0 times the increase in serum []. Absence of compensation in the setting of metabolic alkalosis constitutes the coexistence of a secondary respiratory disturbance.
The third line of defense is renal excretion. This can be described as follows: The normal kidney has a powerful protective mechanism against the development of significant increases in ECF [], namely the plasma threshold for [
], above which proximal reabsorption fails and
losses in urine begin. Mathematically, the plasma [
] can be estimated at a given time following addition of
to the body by:
where []t is the plasma bicarbonate at time t, [
]PT is the plasma threshold for bicarbonate, [
]t0 is the bicarbonate concentration after adding bicarbonate to the body, GFR is the glomerular filtration rate, Vd is the volume of distribution for bicarbonate, and exp is the exponential function.
In nonmathematical terms, once the plasma threshold (PT) is exceeded, bicarbonate excretion in urine is proportional to the glomerular filtration rate (GFR). If a patient has a GFR of 100 mL/min and the bicarbonate concentration is 10 mEq/L above the PT, bicarbonate will be lost in the urine initially at a rate of 1 mEq/min! Therefore, the corrective response by the kidney to excrete excessive in urine usually corrects metabolic alkalosis unless there is a maintenance factor that prevents this. Exceptions to this rule occur when renal function is dramatically impaired and/or when the ongoing alkali load truly overwhelms the renal capacity for bicarbonate elimination. These exceptional situations are both uncommon and easily identified. Therefore, we usually approach the pathophysiology of metabolic alkalosis by addressing initiation factors (ie, factors that initiate the process) and maintenance factors (those that prevent renal excretion of excess bicarbonate). In some cases, as will be seen, the same factor may be responsible for both initiation and maintenance.
KEY POINTS
THE MAINTENANCE OF METABOLIC ALKALOSIS
A number of factors increase the apparent tubular maximum concentration (Tmax) for . As a result, they increase net
reabsorption by the kidney. Figure 8.1 shows this schematically.
FIGURE 8-1. The importance of maintenance factors in the pathophysiology of metabolic alkalosis. In this figure, we see that proton loss (eg, from vomiting) leads to increases in pH and []. These increases in [
] are accompanied by increases in
filtration and loss in urine. If a maintenance factor (eg, volume depletion, primary mineralocorticoid excess) is present, however, that raises the tubular transport of
(Tmax), increased renal losses of
are prevented, and metabolic alkalosis is maintained. Note that the higher pH causes a decrease in alveolar ventilation (VA; see Chapter 9) and the PaCO2 increases.
Arterial Blood Volume Decrease
Volume depletion either absolute (eg, salt losses through vomiting or bleeding) or effective (eg, congestive heart failure, nephrotic syndrome, hepatic cirrhosis) increases the Tmax and PT for . This occurs through both proximal (increased proximal tubule reabsorption of Na+ and water) and distal (mineralocorticoid effect) mechanisms. Catecholamines and angiotensin II stimulate the Na+-H+ exchanger isoform in the luminal membrane of proximal tubule (NHE3). Proton excretion into urine generates bicarbonate that is transported across the basolateral membrane into blood. Mineralocorticoids act distally to directly stimulate the H+-ATPase, and indirectly raise the driving force for proton excretion by increasing lumen electronegativity (through stimulation of the epithelial sodium channel).
Chloride Depletion
Sodium and chloride losses result in ECF volume depletion. Studies show that chloride is independently (ie, besides being a marker for ECF volume) involved in reabsorption. In fact, even despite ECF expansion, chloride depletion increases the PT for
, thereby raising ECF [
].
Aldosterone
Mineralocorticoids increase distal sodium reabsorption which, in turn, increases renal generation and effectively raises the PT and Tmax for
. These effects can occur in the absence of decreases in effective arterial blood volume. Aldosterone’s predominant effect is in distal nephron. Figure 8.2 shows a model of 2 of the 3 major cell types in the collecting duct, the principal cell and the α intercalated cell. The principal cell is responsible for sodium reabsorption and potassium secretion. The α intercalated cell mediates acid secretion and, therefore, bicarbonate reabsorption and generation. Potassium secretion is passive and dependent strictly on the electrochemical gradient. Potassium secretion can be increased by raising intracellular potassium, lowering luminal potassium, or making the lumen more electronegative. Indeed, the major factors that control distal potassium secretion operate by changing these driving forces. Stimulation of the Na+-K+-ATPase by aldosterone, increases intracellular potassium. Aldosterone also increases distal sodium reabsorption by causing the insertion of sodium channels, as well as synthesis of new sodium channels. In the long term, aldosterone also increases the expression of the Na+-K+-ATPase in most epithelial cells, and directly stimulates the H+-ATPase present in the luminal membrane of the intercalated cell. It also acts indirectly by increasing lumen electronegativity (through sodium reabsorption). Aldosterone binds to its receptor in the cytoplasm; this complex then translocates to the nucleus and stimulates gene transcription.
FIGURE 8-2. Collecting duct cell model. Proteins involved in sodium, potassium, and acid–base homeostasis are shown in both principal cells and α intercalated cells.
Surprisingly, it was found that glucocorticoids have similar affinity to that of aldosterone for the mineralocorticoid receptor. In addition glucocorticoids circulate at many times the concentration of aldosterone. So how could aldosterone ever have an effect? The answer to this question lies in the fact that target tissues for aldosterone, such as collecting duct cells, possess the enzyme type II 11β-hydroxysteroid dehydrogenase (HSD) that degrades active cortisol to inactive cortisone. If this enzyme is congenitally absent (apparent mineralocorticoid excess), inhibited (licorice), or overwhelmed (Cushing syndrome), then glucocorticoids can exert a mineralocorticoid-like effect in collecting duct.
Potassium Depletion
Potassium depletion also may increase the apparent Tmax and PT for and, thus, act as a maintenance factor for metabolic alkalosis. One potential mechanism for this is that potassium depletion may promote a relative intracellular acidosis, which makes renal H+ excretion more favorable; however, there is considerable evidence against this appealing concept. For one, there are orders of magnitude in concentration differences involved when we compare protons to potassium ions. The [H+] in ECF is only approximately 40 nM (although intracellular concentrations may be slightly higher), whereas potassium concentrations may change by 1 to 2 mmol/L. More problematic is the observation that investigators failed to detect a decrease in renal intracellular pH during experimental potassium depletion with 31P nuclear magnetic resonance (NMR) spectroscopy. Moreover, in human studies, metabolic alkalosis can be corrected almost completely without correction of potassium depletion. More likely mechanisms for the increased Tmax for
resulting from K depletion follow. First, potassium depletion results in cellular potassium depletion in the proximal tubule. This, in turn, would be expected to hyperpolarize the basolateral membrane and increase the driving force for bicarbonate exit via the Na+-3
cotransporter. Second, potassium depletion upregulates H+-K+-ATPase in the collecting duct’s intercalated cell. It is likely that this upregulation results in increased H+ secretion in this segment. This, in turn, would result in
generation and addition to ECF.
Hypercapnia
The apparent Tmax and PT for are raised by increases in PaCO2. This is probably related to the decreases in intracellular pH that occur during acute and chronic hypercapnia. Analogous to our discussion in Chapter 7, increases in PaCO2 that occur during metabolic alkalosis as part of normal respiratory compensation impair the ability of the kidney to return serum bicarbonate concentration to normal.
KEY POINTS
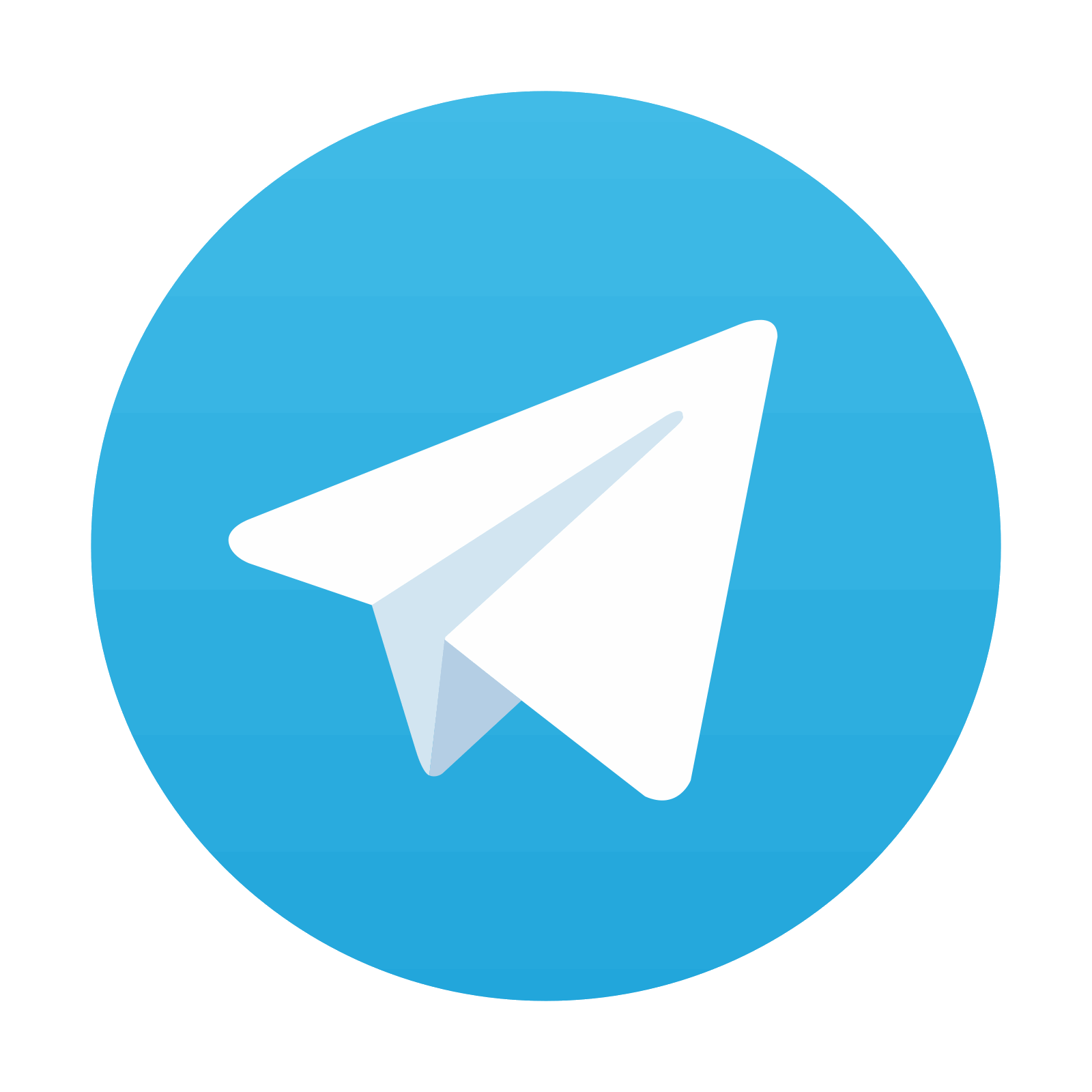
Stay updated, free articles. Join our Telegram channel
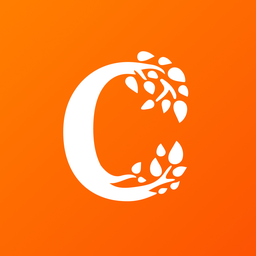
Full access? Get Clinical Tree
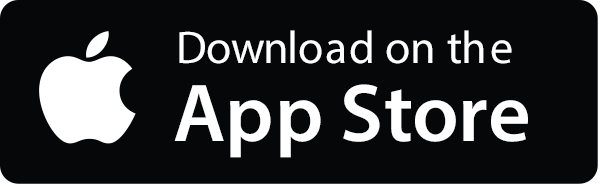
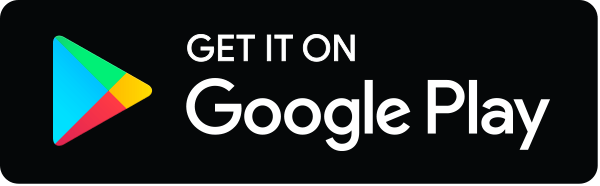