Metabolic acidosis
The “secrets” of metabolic acidosis will be the emphasis of this chapter, given the breadth of this topic. This chapter is parsed into, first, a brief overview of relevant physiology and, second, an algorithmic, clinically relevant approach to the metabolic acidosis. The final section of the chapter will discuss, in more detail, specific examples of this diverse group of metabolic abnormalities.
Background and key physiology
1. The difference between acidemia and acidosis.
Acid emia describes the concentration of hydrogen ions ([H + ]) in plasma; thus it includes all conditions where [H + ] in plasma is higher than the values observed in normal subjects (40 ± 2 nmol/L, or a pH less than 7.38). Acid emia has two general causes, and this leads to the concept of acid osis . An acid osis is any process by which there is increased [H + ] and a decrease in the concentration and/or the content of bicarbonate (HCO 3 − ) in the extracellular fluid (ECF) compartment. As noted previously, there are two general processes whereby [H + ] is increased in plasma. First, there may be a high carbon dioxide tension in arterial blood (i.e., the PaCO 2 —called a respiratory acidosis) or, second, a high [H + ] in plasma from a low bicarbonate (called metabolic acidosis). Notably, an acidosis does not necessarily mean there is an acidemia. This is because there may be multiple processes—of metabolic or respiratory origin—that cause [H + ] in plasma to fall despite there being an identified metabolic acidosis.
2. What is metabolic acidosis?
A metabolic acidosis is a metabolic process resulting in increased [H + ]; there are two main etiologies:
- A.
The addition of an acid ( Fig. 78.1 , shift in equation 1 from left to right)
Figure 78.1.
Buffer systems to remove a H + load.
Although equations 2 and 3 describe buffer systems to remove H + in terms of chemistry of this process, there is a different emphasis in physiologic terms. In this context, flux through equation 2 must be minimized. This occurs if these added H + are forced to bind to bicarbonate (HCO 3 − ) by lowering the PCO 2 in capillaries of skeletal muscles (equation 3) where this buffer system is most abundant, thereby pulling equation 3 to the right, which lowers the concentration of H + in plasma and minimizes binding of H + to intracellular proteins in vital organs (e.g., brain and heart).
- B.
The loss of HCO 3 , usually with sodium (Na + ) (NaHCO 3 ), or potassium (K + ), or both (see Fig. 78.1 , shift in equation 3 from right to left).
3. What risks does metabolic acidosis entail for the patient?
The risks that metabolic acidosis pose to the patient arise from multiple mechanisms:
- •
There is risk engendered by the underlying disorder responsible for the metabolic acidosis (e.g., shock).
- •
There are the adverse effects of high [H + ] (binding of H + to intracellular proteins in vital organs (e.g., brain and heart; see equation 2 in Fig. 78.1 ), as well as possible dangers associated with the anions that accompany the H + load (e.g., chelation of ionized calcium during citric acidosis).
- •
There are risks from toxins produced in the metabolic process that lead to the production of these acids (e.g., formaldehyde produced during the metabolism of methanol to formic acid or glycoaldehyde produced during metabolism of ethylene glycol to glycolic acid) or as a marker of a serious metabolic threat (e.g., production of pyroglutamic acid after overdoses of acetaminophen, as this indicates depletion of the reduced form of glutathione—the protein that scavenges reactive oxygen species).
4. What are the benefits of metabolic acidosis?
It should also be recognized that there may be benefits from metabolic acidosis. Most importantly, the Bohr effect leads to a shift of the hemoglobin-O 2 dissociation curve to the right, leading to more unloading of O 2 to tissues. Whatever adverse effects occur as the result of acidosis, treatment of metabolic acidosis is not necessarily beneficial, particularly when acute. Administration of sodium bicarbonate may not lead to the expected benefit and can itself lead to adverse effects.
5. What is the bicarbonate buffer system (BBS)?
To minimize binding of H + to intracellular proteins, another process must prevent [H + ] from rising, despite the H + load. This occurs if added H + are forced to bind to bicarbonate (HCO 3 − ), the predominant extracellular buffer (see Fig. 78.1 , equation 3), and this illustrates the BBS. The vast majority of HCO 3 − is in skeletal muscle. If the BBS in skeletal muscle is not appropriately titrating, and therefore removing H + in a patient with metabolic acidosis, acidemia will be more pronounced, and more of the H + load will be titrated in vital organs (e.g., brain and heart; Fig. 78.2 ). Critically, for the BBS to function there must be a low concentration of carbon dioxide (CO 2 ) in capillaries around skeletal muscle; in other words, equation 3 must maintain its rightward direction (i.e., Fig. 78.1 , equation 3).

6. How do you assess the adequacy of the BBS?
A high tension of capillary CO 2 within skeletal muscle will lead to a failure to maintain rightward shift (equation 3 in Fig. 78.1 ), thus extinguishing the BBS. CO 2 tension within muscle capillaries can be assessed with a venous blood gas (VBG). This is because the PCO 2 in capillaries of skeletal muscle reflects the PCO 2 in its cells and interstitial space; further, there is no CO 2 added once the blood leaves the capillaries and enters the veins. The secret here is that if the partial pressure of carbon dioxide in the venous effluent (PvCO 2 ) is high, the BBS in skeletal muscle is less effective (i.e., the rightward direction of equation 3 is blunted). Acidemia will be more pronounced, as more of the H + load will be titrated in vital organs.
7. What are the major reasons for a high PvCO 2 ?
The PvCO 2 is essentially the summation of the arterial PCO 2 (PaCO 2 ) entering the capillaries and the CO 2 added by cellular metabolism. At the usual blood flow rate at rest, this results in a brachial PvCO 2 of 46 mm Hg, assuming a normal input arterial PaCO 2 of 40 mm Hg. Accordingly, the reasons for a high PvCO 2 are due to either a high PaCO 2 (i.e., respiratory suppression/failure), which raises the lowest possible value for PvCO 2 , and/or a slow blood flow rate to muscle (e.g., poor perfusion states such as evolving shock). The reason that diminished tissue perfusion raises PvCO 2 is outlined as follows:
- 1.
When capillary blood flow falls, tissue oxygen consumption remains constant.
- 2.
Constant oxygen consumption leads to constant CO 2 production (as related by the respiratory quotient; i.e., 0.7 to 1.0 mmol of CO 2 will be formed per mmol of O 2 consumed).
- 3.
Despite constant CO 2 production, in the context of low flow, there is relatively more CO 2 added to each liter of blood volume; consequently, PvCO 2 rises.
Clinically relevant approach to a patient with metabolic acidosis
8. What is the general approach to metabolic acidosis evaluation?
Metabolic acidosis can be thought of as comprising two major subgroups: one underpinned by the addition of [H + ] and the other driven by the loss of NaHCO 3 . The distinction here is generally guided by the calculation of the anion gap. Prior to this, however, it is prudent to identify and manage underlying threats to the patient’s life consequent to the acidosis. In addition, therapy for the acidosis may unmask adverse effects that should be anticipated. Examples will be considered later on in this chapter, during the discussion of specific disorders. Lastly, when able, assess the adequacy of the BBS by comparing the arterial and venous tensions of CO 2 . Therapy directed at lowering arterial PaCO 2 should be instituted if there is concomitant respiratory failure, and tissue perfusion should be enhanced if there is a gradient (i.e., more than 10 mm Hg) between the PaCO 2 and PvCO 2 . As noted previously, lowering the venous and therefore capillary CO 2 shifts equation 3 rightward. In this way, bicarbonate rather than tissue proteins buffer the excess protons. The initial steps in our clinical approach to the patient with metabolic acidosis are illustrated in Fig. 78.3 .

9. How do you calculate the anion gap?
The presence of new anions in plasma can be detected with the calculation of the P Anion gap .
Electroneutrality requires that Na + + unmeasured cations (UC) = Cl − + HCO 3 − + unmeasured anions (UA). Actually, almost everything is measured, but for simplicity’s sake we pretend that the potassium, calcium, phosphate, and others are not.
Then, rearranging, Na + – Cl − – HCO 3 − = UA – UC = anion gap. Assuming some usual normal values, 140 – 104 – 24 = 12 (normal range 6 to 12). The major UA is albumin—about 3 mEq negative charge for each g/dL. So with normal albumin concentration of 4.0 g/dL, it contributes 12 mEq/L, roughly equal to the anion gap. From that equation, it can be seen that an increase in the anion gap is due to an increase in the UA (e.g., lactate) and/or a decrease in the UC (e.g., globulins).
Accordingly, the baseline value of the P Anion gap must be adjusted for the P Albumin . A rough guide for correcting the baseline value of P Anion gap for P Albumin is that at P Albumin of 4.0 g/dL (40 g/L), the P Anion gap is 12 mEq/L; hence for every 1.0 g/dL (10 g/L) decrease in P Albumin , the P Anion gap will be lower by ∼3 mEq/L. The converse is true for a rise in the P Albumin ( Box 78.1 ) .
pH: 7.40 ± 0.02
[H+]: 40 ± 2 nmol/L
[HCO 3 − ] in plasma (PHCO3): 25 ± 2 mmol/L
Arterial PCO 2 : 40 ± 2 mm Hg
Brachial venous PCO 2 : <10 mm Hg higher than the arterial PCO 2 .
Albumin in plasma (P Albumin ): 4.0 g/dL or 40 g/L. Accounts for ∼12 mEq/L of the anionic charge in plasma.
Anion gap in plasma (P Anion gap ): 12 ± 2 mEq/L (must adjust for the valence from the PAlbumin)
Henderson equation: [H + ] (nmol/L) = PCO 2 (mm Hg) × (25 or 24)/ PHCO3
To make the mathematics easier at the bedside, one can use 25 or 24 as the constant.
10. In a patient with an increased anion-gap metabolic acidosis, what is the cause of overproduction of acids?
A list of the causes of metabolic acidosis is provided in Box 78.2 , and a clinical approach to the diagnosis of the cause of metabolic acidosis is summarized in Fig. 78.4 .
Acid gain
With retention of anions in plasma:
- 1.
l -lactic acidosis
- A.
Due predominantly to overproduction of l -lactic acid
Hypoxic lactic acidosis
Inadequate delivery of O 2 (cardiogenic shock, shunting of blood past organs [e.g., sepsis], or excessive demand for oxygen [e.g., seizures])
Increased production of l -lactic acid in absence of hypoxia
Overproduction of NADH and accumulation of pyruvate in the liver (e.g., metabolism of ethanol plus a deficiency of thiamin)
Decreased pyruvate dehydrogenase activity (e.g., thiamin deficiency, inborn errors of metabolism)
Compromised mitochondrial electron transport system (e.g., cyanide, riboflavin deficiency, inborn errors affecting the electron transport system)
Excessive degree of uncoupling of oxidative phosphorylation (e.g., phenformin)
- B.
Due predominantly to reduced removal of l -lactate: liver failure (e.g., severe acute viral hepatitis, shock liver, drugs)
- C.
Due to a combination of reduced removal and overproduction of l -lactic acid
Antiretroviral drugs (inhibition of mitochondrial electron transport plus hepatic steatosis)
Metastatic tumors (especially large tumors with hypoxic areas plus liver involvement)
- A.
- 2.
Ketoacidosis (diabetic ketoacidosis, alcoholic ketoacidosis, hypoglycemic ketoacidosis including starvation, ketoacidosis due to a large supply of short-chain fatty acids [i.e., acetic acid from fermentation of poorly absorbed carbohydrate plus inhibition of acetyl-coenzyme A carboxylase])
- 3.
Kidney insufficiency (metabolism of dietary sulfur-containing amino acids and decreased kidney excretion of NH 4 + )
- 4.
Metabolism of toxic alcohols (e.g., formic acid from metabolism of methanol, glycolic acid, and oxalic acid from metabolism of ethylene glycol)
- 5.
d -lactic acidosis (and other organic acids produced by gastrointestinal bacteria)
- 6.
Pyroglutamic acidosis
With a high rate of excretion of anions in urine:
- 1.
Glue sniffing (hippuric acid overproduction)
- 2.
Diabetic ketoacidosis with excessive ketonuria
NaHCO 3 loss
Direct loss of NaHCO 3 :
- 1.
Via the GI tract (e.g., diarrhea, ileus, fistula)
- 2.
Via the urine (proximal renal tubular acidosis or low carbonic anhydrase II or IV activity)
Indirect loss of NaHCO 3 (low urinary NH 4 + secretion):
- 1.
Low glomerular filtration rate
- 2.
Renal tubular acidosis
- A.
Low availability of NH 3 (urine pH ∼5) = problem in PCT ammoniagenesis: hyperkalemia, alkaline pH in PCT cells
- B.
Defect in net distal H + secretion (urine pH often ∼7):
H + ATPase defect or alkaline α-intercalated cells (a number of autoimmune disorders or disorders with hypergammaglobulinemia; e.g., Sjögren syndrome)
H + back-leak (e.g., amphotericin B)
HCO 3 secretion in the collecting ducts (e.g., a molecular defect in the Cl-/HCO 3 − anion exchanger leading to its mistargeting to the luminal membrane of α-intercalated cells, as in some patients with Southeast Asian ovalocytosis)
- C.
Problem with both distal H + secretion and medullary NH 3 (urine pH <6): Diseases involving the kidney interstitial compartment (e.g., sickle cell disease)
- A.
PCT , Proximal convoluted tubule.

A secret heuristic for the causes of an elevated anion gap is “KULT”:
- •
Ketones (diabetic, fasting and alcoholic ketoacidosis)
- •
Uremia (with retained phosphate and sulfate and others)
- •
Lactate (e.g., with hypoxia and septic shock)
- •
Toxic ingestions (especially ethylene glycol and methanol)
While ketones are typically detected from the clinical history, a secret is that ketonuria may not be detected on a standard urinalysis if there is a high NADH/NAD ratio (e.g., contaminant, ethanol intake). The reason is that beta-hydroxybutyrate (BHB) is favored when NADH is abundant, and BHB is not detected by the nitroprusside test, which is the urinalysis-based assay. The next test to be sought is the venous lactate measurement. When a VBG is available, also take note of the PvCO 2 , as noted previously. Lastly, consider assessing for toxic ingestions by calculating a serum osmolal gap (P Osmolal gap ). This will determine whether new uncharged particles are present in plasma:
However, as the toxic alcohols ethylene glycol and methanol are metabolized to oxalate and formic acid, the osmolal gap falls as the anion gap rises. Early after ingestion, before metabolism has occurred, the osmolal gap is present. Subsequently, metabolism leads to a rising anion gap such that the time of the peak for both anion and osmolal gaps may not coincide ( Table 78.1 ).
QUESTION | PARAMETER ASSESSED | TOOLS TO USE |
---|---|---|
Is the content of HCO 3 − low in the ECF? | ECF volume | Hematocrit or total plasma proteins |
Have new acids accumulated? | Appearance of new anions in the body or the urine | P A nion gap Urine anion gap |
Are toxic alcohols present? | Detect alcohols as unmeasured osmoles | P Osmolal gap |
Is the renal response to acidemia adequate? | Examine the rate of excretion of NH 4 + | Urine osmolal gap |
If NH 4 + excretion is high, which anion was excreted? | GI loss of NaHCO 3 Acid added, but its new anions are excreted | Urine Cl − is high Urine anion gap |
What is the basis for a low rate or excretion of NH 4 + ? | Low net distal H + secretion Low NH 3 availability Both defects | Urine pH >6.5 Urine pH ∼5.0 Urine pH ∼6.0 |
Where is the defect in H + secretion? | Distal H + secretion Proximal H + secretion | PCO 2 in alkaline urine FE HCO3 , U Citrate |
11. Is there a reproducible quantitative relationship between the fall in [HCO 3 − ] and the rise in the P anion gap in all patients with metabolic acidosis?
The relationship between the rise in the P Anion gap and the fall in the concentration of bicarbonate [HCO 3 − ] is used to detect the presence of coexisting metabolic alkalosis in a patient with metabolic acidosis (i.e., the rise in P Anion gap is larger than the fall in [HCO 3 − ]) and/or the presence of both an acid overproduction type and a NaHCO 3 loss type of metabolic acidosis (i.e., the rise in P Anion gap is smaller than the fall in [HCO 3 − ]). It must be recognized, however, that this relationship uses concentration rather than content terms and therefore will underestimate the magnitude of the HCO 3 − deficit if there is significant contraction of the ECF. That is, diminished ECF volume will suggest an underlying alkalosis (i.e., a process that is increasing the content of bicarbonate), when in fact there is none. This carries clinical relevance in bicarbonate-wasting metabolic acidosis, because the provision of bicarbonate-free fluid resuscitation may lead to a rapid, unexpectedly large fall in [HCO 3 − ] and unmask a severe metabolic acidosis, described as follows under specific disorders (e.g., diarrhea; see Table 78.1 ).
12. Estimate the “corrected” bicarbonate concentration when there is significant ECF volume contraction.
The hematocrit or total protein levels in plasma may be used to obtain a quantitative estimate of the ECF volume.
Sample calculation: If the patient does not have preexisting anemia or polycythemia, use the hematocrit (though the concentration of albumin or total proteins in plasma may also be used) to obtain a quantitative estimate of the ECF volume and calculate its content of HCO 3 − . In a normal adult, the usual hematocrit is 0.40 (40%); this represents a blood volume of 5 L (2 L of red blood cells [RBC] and 3 L of plasma):
Therefore when the hematocrit is 0.50, the plasma volume equals the of RBC volume (i.e., 2 L):
The present blood volume is 4 L, and thus the plasma volume is 2 L. Hence the plasma volume is reduced by 1 L from its normal value of 3 L. Ignoring changes in Starling forces for simplicity, the ECF volume is diminished by ∼33%; this implies that rapid restoration of ECF volume would lead to a [HCO 3 − ] that is 33% of its measured value.
13. In a patient without an elevated anion gap, with a hyperchloremic metabolic acidosis, estimate the urinary excretion of NH 4 + .
If increased protons are added to the circulation with the “unmeasured” anions discussed previously, an increase in the anion gap occurs. But if such anions are not present, added protons will appear to have been added as hydrochloric acid, HCl. This is because when protons accumulate and serum bicarbonate falls, the kidney will have to reabsorb Na + with Cl − , out of proportion to normal [Cl − ]. The result is that the anion gap does not change and hyperchloremic acidosis ensues. A major example is renal tubular acidosis (RTA), where the kidney fails to excrete protons. The loss of HCO 3 − in the stool, with diarrhea, is another example. Again, Na + is reabsorbed in the kidney with Cl − , and hyperchloremic acidosis occurs. For practical purposes, hyperchloremic metabolic acidosis is due either to diarrhea or RTA. Other disorders that lead to hyperchloremic metabolic acidosis generally fall into one of these categories.
In the context of a chronic hyperchloremic metabolic acidosis and normal renal function, the urinary excretion NH 4 + of should rise, and can exceed 200 mmol/day. Most laboratories do not measure U NH4 (without good reason); therefore one way to indirectly estimate U NH4 is the urine osmolal gap (U Osmolal gap ). Because NH 4 + is excreted with an anion, U NH4 is half the calculated value of U Osmolal gap .
To convert U NH4 into an excretion rate, divide it by the concentration of creatinine in the urine (U Creatinine ). If the ratio of U NH4 /U Creatinine is considerably <40 mmol/g creatinine (or <4, in mmol/mmol terms), a low rate of excretion of NH 4 + is likely to be an important cause of the acidemia; such pathophysiology is a hallmark of RTA.
14. In a patient without an elevated anion gap (with hyperchloremic metabolic acidosis) and a low rate of excretion of NH 4 + , what is the basis for a low NH 4 + excretion rate ( fig. 78.5 )?
The urine pH is most valuable to identify the pathophysiology of the low rate of excretion of NH 4 + . If the urine pH is greater than 6.5, the low rate of excretion of NH 4 + is likely the result of a reduced net rate of distal H + secretion. In contrast, if the urine pH ∼5, the low rate of excretion of NH 4 + is usually the result of a disease, leading to a diminished production of NH 4 + in the proximal convoluted tubule (PCT). However, if urine pH is ∼6, there will be a defect that lowers the availability of both H + and NH 3 in the lumen of the medullary collecting duct.

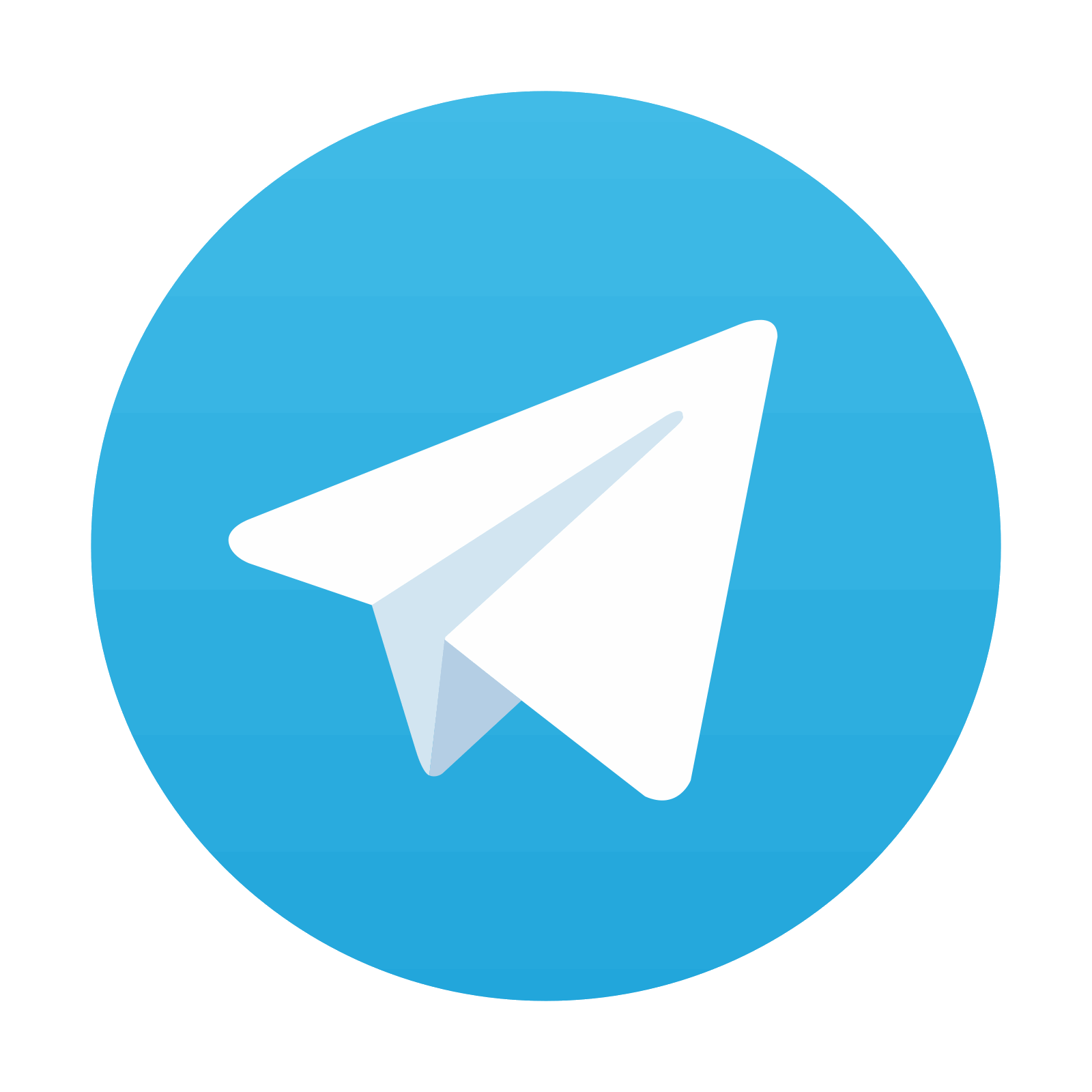
Stay updated, free articles. Join our Telegram channel
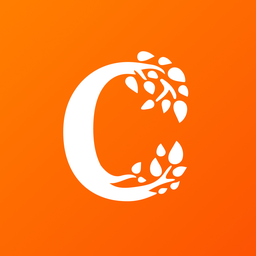
Full access? Get Clinical Tree
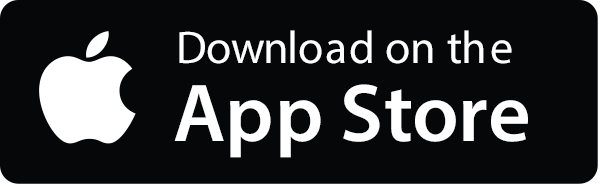
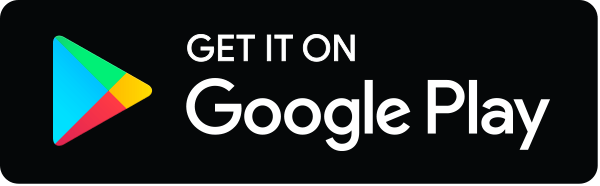
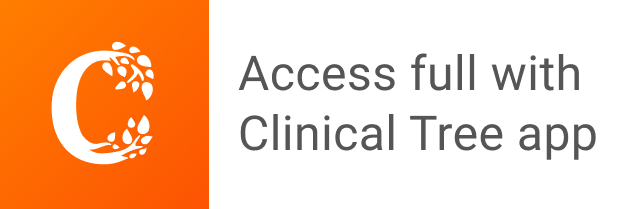