ACID–BASE CHEMISTRY AND BIOLOGY
Acid–base disorders are one of the most common problems encountered by the clinician. Although the degree of acidosis or alkalosis that results is rarely life threatening, the careful evaluation of the patient’s acid–base status often provides insight into the underlying medical problem. Moreover, the pathophysiology and differential diagnosis of these disorders can be approached logically with a minimum of laboratory and clinical data. Without exaggeration, we would say that analysis of the acid–base disturbance makes one’s clinical approach to a patient substantially easier. In Chapters 7 to 9, we summarize the “physiologic approach” to acid–base disorders, which we believe is simple, logical, and clinically relevant. Acid–base homeostasis consists of the precise regulation of CO2 tension by the respiratory system and plasma bicarbonate () concentration [
] by the kidney. The kidney regulates the plasma [
] by altering
reabsorption and elimination of protons (H+). The pH of body fluids is determined by CO2 tension and [
]. These body fluids can generally be readily sampled and analyzed with a blood gas instrument that determines CO2 tension (in arterial blood, PaCO2), pH, and [
], the latter that is generally calculated (see below). Primary abnormalities of CO2 tension are considered respiratory disturbances, whereas primary derangements of [
] are referred to as metabolic disturbances.
Understanding clinical acid–base chemistry requires an appreciation of buffers. For diagnostic purposes, we can define an acid as a chemical that donates a H+, and a base as a H+ acceptor. For an acid (HA) and its conjugate base (A–), we describe its strength (or tendency to donate a H+) by its dissociation constant Keq and the formula:
If we rearrange this equation and apply a log transformation, we arrive at the following:
We use the term buffering to describe the capacity of a solution to resist a change in pH when a strong (ie, highly dissociated) acid or alkali is added. As a concrete example, say we added 100 mL of 0.1 M HCl to 900 mL of distilled water. The [H+] of what was previously distilled water would increase from 10–7 to 10–2 M. In other words, the pH would fall from 7.0 to 2.0. In contrast, if we added 100 mL of 0.1 M HCl to 900 mL of a 1 M phosphate buffer (pK = 6.9 at pH 7.0), most of the dissociated H+ from HCl would associate with dibasic phosphate () and the ratio of dibasic to monobasic (
) phosphate would only be slightly changed. As a result, the pH would fall by only 0.1. In this latter example, the hydrochloric acid (HCl) was buffered by the phosphate solution, whereas in the case where HCl was added to distilled water, no such buffering occurred.
In higher animals, such as mammals, the most important buffer in the extracellular space is the bicarbonate buffer system. Inorganic phosphate and proteins are less important buffers in the extracellular space. In the intracellular space inorganic phosphate is quantitatively the most important buffer followed by bicarbonate and intracellular proteins (Figure 7.1). Although intracellular pH (pHi) is probably more important in predicting physiologic and clinical consequences than extracellular pH, it is extremely difficult to measure in vivo. Because extracellular acid–base status is still informative, we focus our clinical efforts on classifying disease states using this information, which can readily be obtained. Specifically, we focus our attention on the bicarbonate buffer system (Figure 7.1). It is generally assumed that equilibrium conditions apply to the bicarbonate buffer system in blood because of the abundance of carbonic anhydrase (CA) in red blood cells and the high permeability of the red blood cell membrane to components of the bicarbonate buffer system. Therefore, we can express the following equations:
FIGURE 7-1. Relative importance of different buffers in intracellular and extracellular spaces. Note that in the intracellular space, phosphate and proteins play a greater role than they do in the extracellular space, where the bicarbonate buffer system is most important.
or
Furthermore, H2CO3 is defined by the partial pressure of CO2 and the solubility of CO2 in physiologic fluids that is, for all intents and purposes, a constant S. We can, therefore, rearrange Equation 4 to read:
Taking the antilog of both sides we get:
which is called the Henderson-Hasselbalch equation. In blood (at 37°C), the pK referred to in Equation 6 is 6.1 and the solubility coefficient for CO2 (S) is 0.03. Therefore, we can simplify this expression to:
This formula allows us to view acid–base disorders as being attributable to the numerator of the ratio (metabolic processes), the denominator (respiratory processes), or both (mixed or complex acid–base disorders).
KEY POINTS
ASSESSING ACID–BASE BALANCE
A myriad of enzymatic reactions involve the loss or gain of protons that occur with ongoing catabolism and anabolism. To understand whether acid or base is produced, however, one simply examines the initial substrates and final products. To do this, it is helpful to think of acids and bases as “Lewis” acids and bases; in other words, to consider acids as electron acceptors rather than as proton donors. In concrete terms, when a substrate is metabolized to something more anionic (eg, glucose is metabolized to lactate through the Embden-Meyerhof glycolytic pathway), acid is generated. Conversely, if a substrate is metabolized to something more cationic (eg, lactate is metabolized to CO2 and H2 O via the tricarboxylic acid [TCA] cycle), acid is consumed (Figure 7.2). Because of the importance of the bicarbonate buffer system in overall acid–base homeostasis, we generally consider the addition of a proton as equivalent to the decrease in total-body and loss of a proton as a gain in
.
The classic normal values for an arterial blood gas are pH: 7.4; []: 24 mEq/L; and PaCO2: 40 mmHg. The kidneys regulate serum [
] and acid–base balance by reclaiming filtered
and generating new
to replace that lost internally in titrating metabolic acid and externally (eg, from the gastrointestinal tract). Approximately 1 mmol of H+/kg body weight per day is generated from the metabolism of a normal “Western diet.” To maintain acid–base homeostasis, the kidney must excrete this acid load. The role of the kidney in acid–base homeostasis can be divided into 2 basic functions: (a) the reabsorption of filtered bicarbonate; and (b) the excretion of the acid load derived from dietary metabolism.
FIGURE 7-2. “Black box” approach to acid–base metabolism. The left panel shows that when a substrate is metabolized to a more electronegative product, a proton is generated. Conversely, the right panel demonstrates that when a substrate is metabolized to a more electropositive product a proton is consumed and a bicarbonate generated.
KEY POINTS
ACID EXCRETION BY THE KIDNEY
Our understanding of renal acid excretion has evolved considerably in the past decade. In particular, we have identified the specific ion pumps and transporters that are involved in tubular proton secretion in different portions of the nephron. It is clear that the major ion transporters and pumps include the sodium-proton exchanger (Na+-H+ exchanger, which exchanges 1 H+ for 1 sodium ion), the sodium-phosphate cotransporter (which transports 3 sodium ions with 1 molecule), and the vacuolar H+-adenosine triphosphatase (ATPase) (which pumps H+ directly into the tubular lumen). Other important transport proteins include chloride-bicarbonate exchangers, the “colonic” H+ -K+-ATPase, and the Na+-K+-ATPase. These transport proteins are expressed to varying degrees in different cell types and nephron segments of the kidney, depending on the specific functions of these cells.
Regarding overall acid–base handling by the kidney, there is a strong relationship between acid secretion and the reclamation of filtered bicarbonate, as well as the production of new bicarbonate by the kidney as one would anticipate based on our earlier discussion. First, plasma is filtered at the glomerulus and enters the tubular lumen. Each
molecule that is reclaimed requires the epithelial secretion of one H+. This H+ secretion occurs via the Na+-H+ exchanger on the luminal membrane or through an electrogenic H+-ATPase. On an integrated physiologic level, we can think of the
reabsorption processes establishing a plasma threshold for bicarbonate; that is, that level of plasma
at which measurable
appears in urine. This concept of a plasma threshold is well established for renal glucose handling; historically, the appearance of glucose in urine was used as a surrogate for elevated blood glucose levels before blood glucose monitoring became widespread. Continuing this analogy to renal glucose handling, we can also define the maximal net activity of tubular
reabsorption as the Tmax. The Tmax and plasma threshold for
are, of course, intimately related. As the Tmax for
increases, the plasma threshold for
increases. Conversely, decreases in Tmax result in decreases in the plasma threshold. Quantitatively, to eliminate
from urine with a glomerular filtration rate of 100 mL/min and a plasma [
] of 24 mEq/L, the tubules must secrete about 2.4 mmol of H+ per minute. Ergo,
reclamation by the tubules involves a considerable amount of H+ secretion.
Bicarbonate reclamation is closely related to sodium reabsorption and is, therefore, sensitive to a number of other influences that impact sodium reabsorption. In particular, states of extracellular fluid (ECF) volume expansion and decreases in PCO2 decrease the apparent Tmax for , whereas ECF volume contraction and increases in the partial pressure of carbon dioxide (PCO2) increase the apparent Tmax for
. Parathyroid hormone inhibits proximal tubule
reabsorption and lowers the apparent Tmax and plasma threshold for
. The majority of
reabsorption (approximately 80% to 90%) takes place in proximal tubule. The enzyme CA is expressed intracellularly, as well as on the luminal membrane of the proximal tubule cell, which allows the secreted H+ to combine with tubular fluid
to form carbonic acid (H2CO3). This H2CO3 rapidly dissociates to form H2O and CO2, which then can reenter the proximal tubule cell. Intracellularly, water dissociates into H+ and OH–. Intracellular CA catalyzes the formation of
from CO2 and OH–. Bicarbonate leaves the cell via several bicarbonate transport proteins, including the sodium-bicarbonate cotransporter, as well as the Cl––
exchanger. In proximal tubule, where the reclamation of
filtered from the blood occurs,
is formed inside the renal tubular cells when either H+ secretion or ammonium (
) synthesis occurs. The
is then transported back into blood predominantly via the basolateral Na+-3
cotransporter.
Proton secretion by distal nephron is aided by the production of an electrogenic gradient. This gradient, which is produced by removal of sodium from the luminal fluid in excess to anion reabsorption, favors H+ secretion. There is also direct pumping of H+ into the tubular lumen. Na+-H+ exchange, as well as the activities of the vacuolar H+-ATPase and the Na+-K+-ATPase in intercalated and principal cells accomplish these tasks. Chloride exchange with bicarbonate on the basolateral side of these distal tubular cells allows for proton secretion to be translated into bicarbonate addition to blood, as discussed earlier. The epithelial membrane in the distal nephron must not allow backleak of H+ or loss of the electrogenic gradient. Under normal circumstances, urine pH can be as low as 4.4. This represents a 1000:1 gradient of [H+] between the cell and tubular lumen.
Net acid excretion (NAE) is the total amount of H+ excreted by the kidneys. Quantitatively, we can calculate NAE to be the amount of H+ (both buffered and free) excreted in urine minus the amount of and citrate that failed to be reclaimed. Because H+ secretion into the tubule lumen results in a 1:1
addition to the ECF, NAE equals the amount of new
generated.
NAE is accomplished through 2 processes that are historically separated on the basis of a colorimetric indicator (phenolphthalein) that detects pH changes effectively between pH 5 and 8. That acid, which can be detected by titrating sufficient alkali into urine to achieve color changes with this indicator, is called titratable acid and is mostly phosphate in the form. Nontitratable acid excretion occurs primarily in the form of
. This form of acid excretion is not detected by phenolphthalein since the pK (ionization constant of acid; approximately 9) for
is too high. Even though most clinicians equate NAE with an acidic urine, it is important to recognize that a low urine pH does not necessarily mean that NAE is increased. For example, at a urine pH of 4.0, the free H+ concentration is only 0.1 mmol. In a 70-kg person on an average Western diet, one can see that free protons would make up only a small fraction of the approximately 70 mmol of net acid that need to be excreted per day. The majority of NAE is in the form of protons bound to buffers, either phosphate or
. This makes it possible to elaborate a much-less-acid urine but still achieve adequate NAE. In fact, there are several pathologic conditions (discussed later) in which the urine pH is relatively acid but NAE is insufficient. In subjects that consume a typical Western diet, adequate NAE occurs through the hepatic synthesis of glutamine, which is then taken up by the proximal tubule, where it is deaminated to synthesize
(which generates
), as well as H+ and
secretion in the distal and collecting tubules.
NAE is influenced by several factors, including the serum potassium concentration (serum K+ elevations decrease excretion, while decreases enhance distal nephron H+ secretion), PaCO2 (see below), and the effects of aldosterone. Quantitatively, NAE is usually evenly divided between titratable acid and
excretion.
However, our capacity to increase NAE is mostly dependent on enhanced ammoniagenesis and excretion. The older view that
excretion was accomplished by simple passive trapping of
in the tubular lumen has been revised. We now understand that the excretion of
is more “active.” First, hepatic synthesis of glutamine is sensitive to a number of factors including protein absorption from the gastrointestinal (GI) tract. This is probably why patients on total parenteral nutrition may develop metabolic acidosis unless they receive supplemental base-generating substrate such as acetate. In proximal tubule cells, there is deamination of glutamine to form alpha-ketoglutarate (αKG) and two
. The further metabolism of αKG to CO2 and H2O generates 2 new
molecules. Proximal tubule cells actively secrete
into the lumen, probably via the luminal Na+-H+ exchanger.
can substitute for H+ and be transported into the urine in exchange for sodium.
is subsequently reabsorbed in the medullary thick ascending limb of Henle where it can be transported, instead of K+, via the Na+-K+-2Cl– cotransporter. This increases medullary interstitial concentrations of
. Interstitial
enters the collecting duct cell, substituting for K+ on the basolateral Na+-K+-ATPase. The
is next secreted into the tubular lumen, possibly by substitution for H+ in the apical Na+-H+ exchanger or H+-K+-ATPase, and is ultimately excreted into the final urine. Alternatively the nonerythroid proteins RhBg and RhCg may act as
-H+ exchangers in the basolateral and luminal membranes, respectively. It is important to note that the net generation of any
from αKG metabolism is dependent on this excretion of
. Quite simply, if this
molecule is not excreted in urine, it is returned via the systemic circulation to the liver, where it will be used to form urea at the expense of generating 2 H+s. In this case, the
molecules that were generated by the metabolism of αKG are neutralized and no net generation of
results.
Because routine clinical measurement of urinary concentrations never became standard, our appreciation of
in net acid–base balance during pathophysiologic conditions was delayed until surprisingly recently; however, assessment of
is key in understanding NAE. It turns out that urinary [
] is estimated by calculations based on urinary electrolyte concentrations (either urinary anion gap or urinary osmolar gap) that are routinely measured. This is discussed later in this chapter.
KEY POINTS
CLINICAL APPROACH TO THE PATIENT WITH AN ACID–BASE DISORDER
The approach to acid–base disorders often confounds practitioners of medicine; however, if one follows a fairly standard approach, acid–base disorders can be dissected fairly easily. We suggest 7 steps when confronting a suspected acid–base disorder. The information necessary to approach a suspected acid–base disorder involves a blood gas (which gives pH, partial pressure of arterial oxygen [PaO2], PaCO2, and calculated [] values) and serum chemistry panel (which gives serum Na+, K+, Cl–, and total CO2 content). It is these data on which subsequent decisions are based. The total CO2 content (TCO2), which is the sum of the serum [
] and dissolved CO2 (usually determined on a venous serum sample), is often referred to as the “CO2”; however, it must not be confused with the PaCO2, which refers to the partial pressure of CO2 in arterial blood. Since the serum [
] or TCO2 includes a component of dissolved CO2, it is often 1 to 2 mEq/L higher than the calculated [
] derived from arterial blood gases. The 7 steps are:
1. What is the blood pH (is the patient acidemic or alkalemic)? Based on a normal sea level pH of 7.40±0.02, a significant decrease in pH or acidemia means that the primary ongoing process is an acidosis. Conversely, an increase in pH or alkalemia indicates that the primary ongoing process is an alkalosis.
2. What is the primary disturbance? To identify the primary disturbance, one must examine the directional changes of PaCO2 and serum [] from normal. If pH is low and [
] is low, then metabolic acidosis is the primary disturbance. Conversely, if pH is high and [
] is high, then metabolic alkalosis is the primary disturbance.
3. Is compensation appropriate? This step is essential for one to understand whether the disturbance is simple (compensation appropriate) or complex (mixed). With metabolic acidosis, the PaCO2 (in mmHg) must decrease; conversely, with metabolic alkalosis, the PaCO2 must increase. Inadequate compensation is equivalent to another primary acid–base disturbance. It is important to recognize that compensation is never complete; compensatory processes cannot return one’s blood pH to what it was before one suffered a primary disturbance.
4. What is the SAG (discussed in detail later in this chapter)? Calculating the SAG provides insight into the differential diagnosis of metabolic acidosis (anion gap and nonanion gap metabolic acidosis) and can also indicate that metabolic acidosis is present in the patient with an associated metabolic alkalosis.
5. How does the change in SAG compare to the change in serum bicarbonate concentration (discussed more fully in Chapter 9)? If the change in the SAG is much larger than the fall in serum bicarbonate concentration, one can infer the presence of both an anion gap metabolic acidosis and metabolic alkalosis. If the fall in serum bicarbonate concentration is, however, much larger than the increase in the SAG (and the SAG is significantly increased), one can infer the presence of both an anion gap and nonanion gap metabolic acidosis.
6. What is the underlying cause of the disturbance? Identifying the underlying cause of the disturbance is the whole purpose of analyzing acid–base disorders. One must remember that acid–base disorders are merely laboratory signs of an underlying disease. The pathologic cause of the acid–base disorder is usually obvious once the individual primary disturbances are identified.
7. What is the appropriate therapy to initiate? The acid–base disturbance must be directly addressed in several clinical situations. Ultimately, treatment of the underlying cause with the appropriate therapy is most important.
METABOLIC ACIDOSIS
Pathophysiologic Mechanisms and Compensation
Metabolic acidosis is characterized by a primary decrease in []. This systemic disorder may occur in several ways:
1. Addition of a strong acid that consumes .
2. Loss of from the body (usually through the GI tract or kidneys).
3. Rapid addition of nonbicarbonate-containing solutions to ECF, also called dilutional acidosis.
In the latter 2 situations, in which is lost or diluted, an organic anion is not generated. In this case, electroneutrality is preserved by reciprocal increases in serum chloride concentration. These forms of metabolic acidosis are generally referred to as hyperchloremic or nonanion gap metabolic acidosis; however, when an organic acid consumes
, the organic anion that is produced is often retained in ECF and serum. In this circumstance, the serum chloride concentration does not increase. This important concept is discussed in detail below.
The first line of defense against the fall in pH resulting from metabolic acidosis is the participation of buffer systems. This always occurs to some degree. As a general rule, nonbicarbonate buffers buffer about one-half of an acid load; however, with more severe acidosis, the participation of nonbicarbonate buffers can become even more important. Bone contributes importantly to buffering in chronic metabolic acidosis. The attendant calcium loss from bone that results in reduced bone density and increased urinary calcium excretion are major deleterious consequences of chronic metabolic acidosis.
The second line of defense is the respiratory system. The PaCO2 declines in the setting of metabolic acidosis. This is a normal, compensatory response. Failure of this normal adaptive response indicates the concomitant presence of respiratory acidosis. An excessive decline in PaCO2, producing a normal pH, indicates the presence of concomitant respiratory alkalosis. Both situations are considered to be complex or mixed acid–base disturbances (see Chapter 9). The respiratory response to metabolic acidosis is mediated primarily by pH receptors in the central nervous system (CNS). Peripheral pH receptors probably play a smaller role. This explains the small time delay prior to the establishment of respiratory compensation observed in animals and humans subjected to experimental metabolic acidosis. The normal, compensatory fall in PaCO2 (in mmHg) should be between 1 and 1.5 times the fall in serum [] (in mEq/L). Even with extremely severe metabolic acidosis; however, the PaCO2 cannot be maintained below 10 to 15 mmHg.
The kidney provides the third and final line of pH defense. This mechanism is, however, relatively slow compared to the immediate effect of buffering and respiratory compensation, which begins within 30 minutes. In contrast, the renal response requires 3 to 5 days to become complete. In the presence of normal renal function, acidosis induces increases in NAE by the kidney. This increase in NAE is primarily a result of increases in excretion rather than the minimal changes in phosphate (titratable acid) excretion. Acidosis increases the deamination of glutamine that generates
. Excretion of the
and the ultimate catabolism of αKG, leads to generation of new
. In fact, there is both transcriptional and translational upregulation of key enzymes involved in glutamine metabolism that are induced by acidosis. Chronic metabolic acidosis also increases renal endothelin 1 that activates the Na+-H+ exchanger on the proximal tubule brush border. Therefore, acidosis induces both the generation of new
via the glutamine system and the enhancement of
reabsorption and titratable acid formation. Interestingly, the decreases in PaCO2 that occur from respiratory compensation, actually limit renal correction in metabolic acidosis.
KEY POINTS
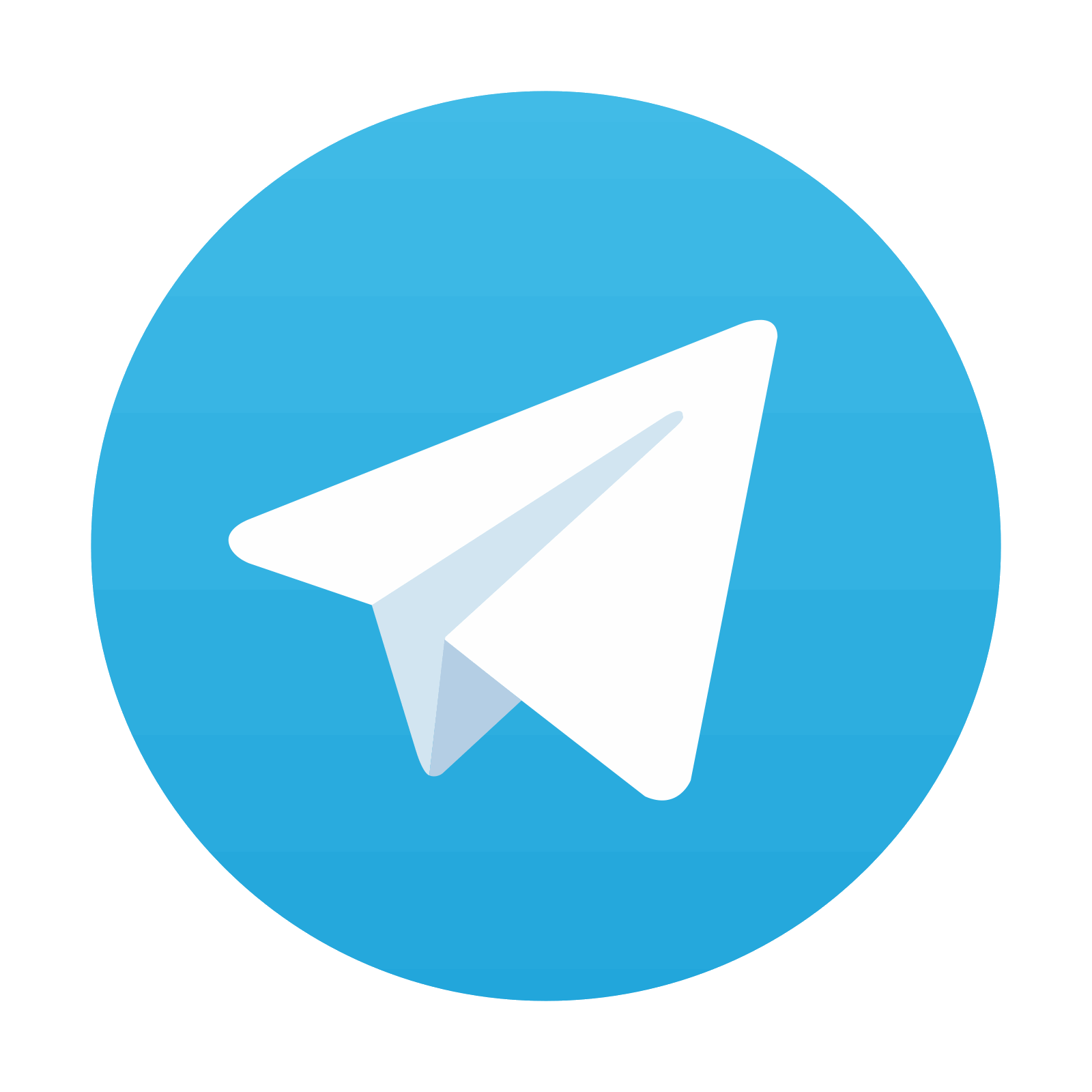
Stay updated, free articles. Join our Telegram channel
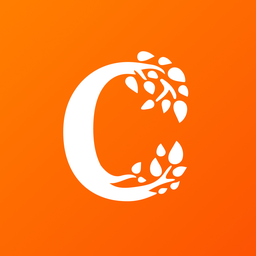
Full access? Get Clinical Tree
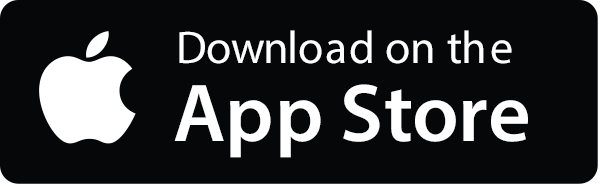
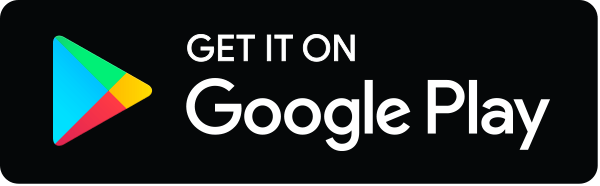