Abstract
In this chapter, we focus on assessment of glomerular filtration rate (GFR) as an index of overall kidney function. The GFR cannot be measured directly. Instead, it is assessed from clearance measurements or estimated from plasma concentrations of filtration markers: exogenous or endogenous solutes eliminated from body fluids primarily by glomerular filtration. Current guidelines by the international group Kidney Disease Improving Global Outcomes (KDIGO) recommend using estimated GFR from creatinine as the “first test” for evaluation of kidney function in routine general practice, with estimated GFR from cystatin C or the combination of creatinine and cystatin C or clearance measurements as confirmatory tests.
Keywords
ACE inhibitors, creatinine, cystatin C, endogenous filtration markers, estimating equation for GFR, estimation of GFR, exogenous filtration markers, glomerular filtration, kidney function, measurement of GFR, urea
Outline
Glomerular Filtration, 23
Measurement of Glomerular Filtration Rate, 25
Physiology of Urinary Clearance and the Measurement of GFR, 25
True GFR versus Measured GFR, 26
Clearance Methods, 26
Exogenous Filtration Markers, 27
Estimation of Glomerular Filtration Rate, 29
Relationship of Glomerular Filtration Rate to Plasma Solute Concentrations, 29
Estimating Equations for Glomerular Filtration Rate, 30
Interpretation of Glomerular Filtration Rate Estimates, 31
Endogenous Filtration Markers, 32
Acknowledgments, [CR]
Acknowledgments
The authors are grateful to Sara Couture, BS, for assistance with manuscript preparation.
The kidney performs specialized functions to maintain constancy of the internal composition of the body fluids. These functions include excretion of waste products, regulation of extracellular fluid volume and composition, production and catabolism of hormones, and regulation of acid–base balance. The normal kidney can adapt to wide variations in intake and in extrarenal loss of fluid and electrolytes through regulation of glomerular filtration and tubular reasbsorption and secretion. In this chapter, we focus on measurement and estimation of glomerular filtration rate (GFR) as an index of overall kidney function. The rationale is that GFR is a property of the kidney, has a large range, and is affected by physiological, pharmacological, and pathological conditions. Furthermore, GFR decline is associated with many physiological and clinical consequences and is correlated with decline in other functions. Decreased GFR is one criterion in the definition and staging of acute and chronic kidney diseases, and GFR estimating equations are recommended for routine use for kidney function assessment in clinical practice.
Glomerular Filtration
Determinants of Glomerular Filtration Rate
The human kidney contains approximately 1 million glomeruli, each approximately 150 to 200 microns in diameter. The total surface area provided for glomerular filtration approximates one square meter. Approximately 180 liters per day (or 125 mL/min) of tubular fluid are produced from renal plasma flow (RPF) by the process of ultrafiltration, driven by the high hydrostatic pressure across the glomerular capillaries and facilitated by the hydraulic permeability of the glomerular capillary wall, which is one to two orders of magnitude greater than other capillaries.
The glomerular filtration barrier is both size- and charge-dependent. Solutes with molecular weights lower than 10,000 daltons freely pass the glomerular capillary wall. Large plasma proteins are excluded from the filtrate as a consequence of the structure of the glomerular capillary wall.
The GFR is dependent on the number of nephrons (N) and the single-nephron glomerular filtration rate (SNGFR), as described here:
GFR = N × SNGFR
In normal individuals and in patients with kidney disease, in whom nephron number may be reduced, regulation of GFR occurs via regulation of SNGFR.
SNGFR = K f ( Δ P − Δ π )
ΔP = the difference between the net transcapillary hydraulic pressure favoring filtration
Δπ = net oncotic pressure opposing filtration
K f = the ultrafiltration coefficient, a composite measure of the surface area and permeability characteristics of the glomerular ultrafiltration barrier
ΔP is determined by the difference between the glomerular capillary hydraulic pressure and that in the earliest proximal tubule. Δπ is determined by the glomerular oncotic pressure alone as the ultrafiltrate is virtually protein-free. Absent from this equation is the RPF rate. Alterations in RPF affect SNGFR largely by influencing ΔP and Δπ.
Variability of Glomerular Filtration Rate
Normal values show considerable variation among individuals, principally due to differences in age, sex, and body size. Hence measured values of GFR are typically adjusted for body size and compared with normative values. A compilation of inulin clearance measurements in young adults shows the mean value in men to be 131 mL/min/1.73 m 2 , and in women to be 120 mL/min/1.73 m 2 ( Fig. 2.1 ), with considerable variation among individuals and over time. Some of these same factors also contribute to variation in GFR in patients with kidney disease.
- 1.
Sex and Body Size. The GFR is related to glomerular surface area and kidney size. Measured values for GFR are conventionally factored by 1.73 m 2 , the mean body surface area (BSA) of men and women 25 years of age. Nonetheless, as described previously, BSA-adjusted values for GFR are approximately 8% higher in young men than in women of the same age. The appropriateness of the use of BSA as the factor by which GFR is adjusted for body size is uncertain. Some have suggested that extracellular volume is a more appropriate index given that the purpose of GFR is to regulate body fluid composition. Adjustment for body size may be of particular importance in obesity, in which GFR is increased, but the increase in body size appears to be proportionately greater than the increase in GFR, potentially leading to a reduction in BSA-adjusted GFR.
- 2.
Age. Both cross-sectional and longitudinal studies show a decline in GFR of approximately 10 mL/min/1.73 m 2 per decade after the age of 30 years, such that during the 50 years from age 30 to age 80, GFR declines by almost 40%, from approximately 130 to 80 mL/min/1.73m 2 . Age-related decline in GFR has been traditionally interpreted as normal; however, other data suggest that there is considerable variation, and recent data suggest that lower GFR in the elderly is associated with increased risk for mortality, which suggests that it is related to disease or other factors rather than normal. This is an area of ongoing debate.
- 3.
Pregnancy. A marked increase in GFR occurs during pregnancy due to an increase in RPF and a decrease in plasma oncotic pressure. GFR may increase up to 50% during the first trimester, persist at that level until term, and then return to normal approximately 4 to 8 weeks after the end of pregnancy. Pregnancy-induced hyperfiltration also occurs in women with preexisting chronic kidney disease, and the percentage increase appears proportionate to the prepregnancy level of GFR.
- 4.
Protein Intake. The effect of protein intake on the GFR varies according to the duration of protein feeding (habitual protein intake vs. meat meals or amino acid infusions) and type of protein (animal vs. vegetable or soya protein sources; essential vs. nonessential amino acids). After a meat meal, GFR and RPF rise within an hour and remain elevated for several hours. Similar increases in GFR and RPF were noted in participants fed a high-, medium-, or low-protein diet for 2 weeks. Some studies suggest a greater response to animal than vegetable protein in habitual diets and in response to protein loads. Conversely, long-term malnutrition is associated with reduced kidney size, suggesting structural and hemodynamic alterations. It had been proposed that protein-induced hyperfiltration represents “renal reserve capacity,” which is lost before the reduction in baseline GFR associated with kidney disease. However, it has now been shown conclusively that changes in GFR occur in response to changes in habitual protein intake or meat meals in patients with kidney disease and reduced GFR and in animals with experimental kidney disease.
- 5.
Diurnal Variation. GFR is approximately 10% higher in the afternoon than in the middle of the night, which may be related to the variation in protein intake or hydration during the day, or to transient reductions in GFR associated with exercise.
- 6.
Race and Ethnicity. There are few studies of measured GFR in healthy populations other than Caucasians. In two studies in India, mean measured GFR in kidney donor candidates was lower than prior studies in Caucasians and did not improve after amino acid infusion, which was attributed to their vegetarian diet. In another study in Pakistan, the observed age-associated measured GFR was lower than in prior studies in Caucasians, and lower protein intake was related to lower observed GFR. A recent study comparing US blacks with whites demonstrated no significant difference in measured GFR.
- 7.
Antihypertensive Therapy. The level of GFR remains relatively constant throughout a wide range of blood pressure. Nonetheless, antihypertensive therapy can be associated with reductions in GFR, due, in part, to the effect of lowering blood pressure and, in part, to specific effects of classes of antihypertensive agents. It has long been known that marked reduction in GFR can complicate treatment in patients with severe hypertension and acute or chronic kidney disease, an effect thought to be due to loss or reset of autoregulation due to sclerosis of the renal vasculature from hypertensive injury. The effects of antihypertensive therapy are discussed in Chapter 4 .

Reductions in GFR in acute and chronic kidney disease may result from a decline in the SNGFR from hemodynamic alterations associated with diseases of other organ systems or from a decrease in the nephron number as a consequence of kidney disease. It is hypothesized that a decrease in nephron number is often associated with an increase in SNGFR (hyperfiltration in remnant nephrons). As a result, there may be substantial kidney damage before GFR decreases. As discussed in Chapter 3 , hyperfiltration is hypothesized to be a major contributor to the development and progression of kidney disease, particularly in diabetes. However, as neither nephron number nor SNGFR can be measured in humans, there is much uncertainty about the causes and consequences of hyperfiltration.
Measurement of Glomerular Filtration Rate
The GFR cannot be measured directly. Instead, it is assessed from clearance measurements or estimated from plasma concentrations of filtration markers: exogenous or endogenous solutes eliminated from body fluids primarily by glomerular filtration.
Physiology of Urinary Clearance and the Measurement of GFR
The “gold standard” for the measurement of GFR is the urinary clearance of an ideal filtration marker. The requirements for an ideal filtration marker are:
- 1.
It is freely filtered at the glomerulus. It passes from glomerular capillary blood into the Bowman space unhindered by its size, charge, or binding to plasma proteins.
- 2.
It is not altered during its passage through the nephron. It is not reabsorbed, secreted, synthesized, or metabolized by the tubules.
- 3.
It is physiologically inert. It does not alter the function of the kidney.
The clearance of a solute is defined as the rate at which it is cleared from the plasma per unit concentration. The clearance of solute “x” (Cx) is given by the following equation:
Cx = Ax/Px
Cx = Ux × V/Px
Ux × V = GFR × Px − TRx + TSx
GFR = ( Ux × V − TRx + TSx ) / Px
GFR = Cx − TRx / Px + TSx / Px
GFR = Cx
True GFR versus Measured GFR
As described later, most filtration markers deviate from ideal behavior and clearance measurements are difficult to perform; thus values for measured GFR often contain an element of error, which differentiates it from the physiological or “true” GFR. True GFR, like other physiological properties that cannot be observed directly, can be modeled using the observed measured GFR and estimates of its error. Measurement error is related to both the specific filtration marker used and the clearance method and can be quantified in terms of bias and precision. Bias generally reflects systematic differences from the ideal filtration marker in renal handling, extrarenal metabolism, or assay of the filtration marker. Imprecision generally reflects random error in performance of the clearance procedure or assay of the filtration marker. Precision is assessed by repeated measurement over a short time and under standard conditions to minimize biological variation. Bias is assessed by comparison to an ideal filtration marker and standardized clearance method. Later, we will discuss clearance methods and filtration markers for assessment of GFR.
Clearance Methods
- 1.
Urinary clearance. Urinary clearance is the most direct method for measurement of GFR. Urine concentration of the filtration marker is assayed in a timed urine sample during which the plasma concentration is assayed. GFR is computed according to eq. 4 . This procedure is applicable for both exogenous and endogenous filtration markers.
For an exogenous filtration marker, multiple (two to four) timed urine collections, each of approximately 20 to 30 minutes, are performed after administration of the marker, and the results are averaged. The classic method of Homer Smith includes fasting conditions in the morning, using a continuous intravenous infusion of the marker, multiple clearance periods requiring repetitive blood and urine collections over 3 hours, oral water loading to stimulate diuresis, bladder catheterization to ensure complete urine collection, and careful timing of blood sampling at the midpoint of the urine collection.
As an alternative to continuous intravenous infusion, the exogenous filtration marker may be administered via bolus intravenous injection. This method requires additional blood samples to compute the average plasma concentration as it declines (see later). Subcutaneous bolus administration of the marker allows for slow release of the marker into the circulation, providing more constant plasma levels compared with intravenous bolus. Spontaneous voiding is used in the majority of research studies and clinical practices.
For an endogenous filtration marker, the urinary collection period may be prolonged to avoid the requirement for water loading, and a single plasma sample obtained either at the beginning or the end of the collection period may be assumed to represent the average plasma concentration. A 24-hour urine collection is the method most commonly used in clinical practice, but it is subject to errors in timing and collection of the urine specimen.
- 2.
Plasma clearance. As an alternative to urinary clearance, GFR can be calculated from plasma clearance after a bolus intravenous injection of an exogenous filtration marker computed from eq. 3 , where Ax is the amount of the marker administered and Px is computed from the entire area under the disappearance curve or from 1-compartment or 2-compartment analysis of the slope of the plasma disappearance plot ( Fig. 2.2 ).
FIG. 2.2
Urinary and plasma clearance calculations after a bolus infusion of an exogneous filtration marker.
(A) Urinary clearance. (B) Plasma clearance.
Advantages of this method include the lack of requirement for urinary collection, which is particularly important in populations wherein bladder emptying may be impaired, such as the elderly or children with urinary tract abnormalities. In principle, plasma clearance methods would have greater precision than urinary clearance methods because they eliminate errors in timing of urine collection and incomplete bladder emptying. This has not been extensively tested.
However, there are also several disadvantages to plasma clearance. First, there is a relatively long time (∼5 hours) required to determine the disappearance curve, with an even longer time required in people with very low GFR (8 to 10 hours). Shorter time periods may lead to overestimation of GFR throughout the GFR range. Second, expansion of the extracellular fluid compartment in edematous conditions causes a prolongation of the first compartment of the two-compartment curve and an overestimation of GFR. Third, extrarenal elimination of the filtration marker leads to an overestimation of urinary clearance, which would be more apparent at lower GFR.
3. Nuclear and other imaging. Measurement of GFR by external counting or imaging over the kidneys and bladder using an exogenous isotopic marker is another alternative to urinary clearance. Studies have been done in conjunction with dynamic kidney imaging using 99m Tc-diethylenetriamine pentaacetic acid (DTPA), comparing the percent kidney (and bladder) uptake at a defined time after injection to simultaneously measured GFR by other techniques. Several studies indicate low correlation of 99mTc-DTPA dynamic renal imaging with simultaneous urinary or plasma clearance, especially in people with normal and elevated GFR, reflecting both bias and imprecision. It is premature to recommend external counting or imaging techniques for routine clinical purposes. The main value of dynamic renal imaging would appear to be in determining the function of each of the two kidneys or for use in individuals already undergoing imaging procedures, rather than as a primary method of measuring GFR.
Recently there has been consideration of magnetic resonance imaging (MRI) for measurement of GFR. Several techniques have been evaluated, including assessment of signal intensity within abdominal organs, measurement of the extraction fraction of the agent, and monitoring of tracer intrarenal kinetics. A recent study highlights a potential advantage of dynamic contrast enhanced MRI, which can assess renal blood flow and filtration fraction as well as GFR. However, the agreement between measured GFR assessed by MRI and plasma clearance of iohexol was only moderate. More study is required before MRI technology can be used for GFR measurement in clinical practice.
Exogenous Filtration Markers
Inulin was used as the filtration marker in the classic studies by Homer Smith and remains the gold standard for exogenous filtration marker. There are now a wide variety of exogenous isotopic and nonisotopic filtration markers that are more available and simpler to use than inulin. The properties of inulin and these alternative filtration markers are described later ( Table 2.1 ).
- 1.
Inulin. Inulin, a 5200-dalton, inert, uncharged polymer of fructose, meets all of the criteria for an ideal filtration marker. It is administered as a continuous intravenous infusion with a long interval for equilibration throughout extracellular fluid because of its large molecular radius. However, inulin is difficult to dissolve in aqueous solutions, difficult to measure, and in short supply. Because of these disadvantages, inulin is unsuitable for clinical assessment of GFR; other filtration markers are required.
- 2.
Iothalamate. Iothalamate is commonly administered labeled with radioactive iodine for ease of assay, but it can also be administered in its nonradioactive form and measured using high-performance liquid chromatography (HPLC) or mass spectroscopy methods. The filtration properties are not affected by the radiolabeling. 125 I-iothalamate, widely available in a pure, stable form (half-life of 125 I is 60 days), is bound to protein to a minor degree. Most, but not all, studies comparing urinary clearance of iothalamate with inulin show a small positive bias (overestimation of inulin clearance), likely due to tubular secretion of iothalamate. Iothalamate is generally administered as a bolus subcutaneous injection in urinary clearance procedures, but it can also be administered as bolus intravenous infusion for plasma or urinary clearance or a continuous subcutaneous infusion for urinary clearance.
- 3.
Iohexol. Iohexol is a nonionic radiographic contrast agent that is administered using bolus intravenous injection and can be used for both plasma and urinary clearance. Recently there has been much interest in iohexol as it provides several theoretical advantages over iothalamate. It appears to exhibit neither protein binding nor tubular secretion, extrarenal elimination is minimal, it is stable in biological fluids, its adverse reactions are rare given the small dose (5 mL 300 mg/mL iodine when assayed with a sensitive assay, described later), and it does not require radioactive tags. Plasma clearance of iohexol appears to underestimate urinary inulin clearance, and renal tubular reabsorption of iohexol has been suggested. The major disadvantage of iohexol is the complexity and expense of its assay. High-performance liquid chromatography, requiring a skilled technician and expensive equipment, must be used when low doses of iohexol (e.g., 5 mL of 300 mg/mL iodine) are administered. Other methods include x-ray fluorescence, but that necessitates administration of significantly larger doses of iohexol (10 to 90 mL of 300 mg/mL iodine); capillary electrophoresis; and neutron activation analysis.
- 4.
Ethylene-diaminetetraacetic acid (EDTA). There is extensive European experience with 54 Cr-EDTA in humans. This marker is not commercially available in the United States. The urinary clearance of 54 Cr-EDTA appears to underestimate urinary inulin clearance by 5% to 15% in most, though not all, studies, suggesting tubular reabsorption or protein binding.
- 5.
DTPA. An analog of EDTA, DTPA is usually labeled with 99m Tc and is available in the United States. The advantages of 99m Tc-DTPA include a short half-life (6 hours) that minimizes radiation exposure, the high counting efficiency of 99m Tc, its availability on a daily basis in most nuclear medicine departments, and the convenience of using it to measure GFR at the time of renal imaging studies. DTPA is freely filtered at the glomerulus, with minimal reabsorption by the tubules, but may undergo extrarenal elimination. One disadvantage is dissociation of 99m Tc from the DTPA and protein binding of 99m Tc, leading to underestimation of GFR. Rigorous quality control is necessary to minimize this error, making it more difficult to standardize across centers. The MRI contrast agent gadolinium (Gd)-DTPA has recently been discussed as a novel exogenous filtration marker. There is a highly sensitive novel immunoassay technique for serum and urine Gd. However, there is some concern about the risk of systemic nephrogenic fibrosis due to toxicity of Gd, even at the low levels administered for GFR measurement. The safety of Gd-DTPA and its accuracy and precision have not been thoroughly tested compared with other exogenous filtration markers.
Clearance Methods | Tubular Effects | ||||||||
---|---|---|---|---|---|---|---|---|---|
Marker | Molecular Weight (Daltons) | Protein Binding | Administration | Plasma or Urinary | Secretion | Reabsorption | Extrarenal Elimination | Assay | Serious Adverse Side Effects |
Inulin | 5200 | No | IV bolus | Urinary | None | None | Small | Chemical | None |
Iothalamate ( 125 I or Nonradioactive) | 614 | Small | IV or SC bolus | Plasma a or urinary | Small (?) | None | None | Gamma counter. T½ 60 d/HPLC/mass spectroscopy | Oral KI required to protect the thyroid from 125 I Anaphylatic reaction and contrast nephropathy at higher doses |
Iohexol | 821 | No (?) | IV or SC bolus | Plasma∗ or urinary | None | Small | Small | HPLC MS | Anaphylatic reaction and contrast nephropathy at higher doses |
51 Cr-EDTA b | 292 | Small | IV or SC bolus | Plasma∗ or urinary | None | Small | None | Gamma counter T½ 28 d | |
99 mTc-DTPA c | 393 | Small | IV or SC bolus | Plasma∗ or urinary | ? | Small | None | Gamma counter T½ 6 h | None |
Gadolinium | 157 | Small | IV or SC bolus | Plasma∗ or urinary | ? | ? | RIA | Nephrogenic systemic fibrosis at low GFR |
a Plasma clearances can be calculated only when marker is administered using intravenous route.
b Ethylenediaminetetraacetic acid.
Estimation of Glomerular Filtration Rate
Clearance measurements are difficult to perform in clinical practice. Instead, the level of GFR is usually estimated from the plasma or serum level of an endogenous filtration marker. In this section, we review the relationship of GFR to plasma solute concentrations, and then we focus on specific markers, including creatinine, cystatin C, and urea ( Table 2.2 ).
Tubular Effects | |||||
---|---|---|---|---|---|
Marker | Molecular Weight (daltons) | Generation | Secretion | Reabsorption | Extrarenal Elimination a |
Metabolites | |||||
Creatinine | 113 | Muscle, diet | ++ | None | Gut flora with advanced CKD |
Urea | 60 | Liver, dietary protein | + | +++ | Not described |
Low Molecular Weight Proteins | |||||
Cystatin C | 13,300 | Nucleated cells | Not described | Catabolized | Not described |
β-2 microglobulin | 11,818 | Nucleated cells | Not described | Catabolized | Not described |
β-trace protein | 23,000–29,000 | Choroid plexus, testes, ovaries | Not described | Catabolized | Not described |
Relationship of Glomerular Filtration Rate to Plasma Solute Concentrations
The plasma concentration of solute x reflects the balance of its rate of generation in body fluids (either from endogenous production or exogenous intake) and its rate of elimination from body fluids (either from excretion or metabolism) ( Fig. 2.3 ). In the steady state, the rates of generation and elimination from body fluids are equal and the plasma concentration of solute x is constant, thus the following equation applies.
Gx = Ux × V + Ex

For solutes excreted only in the urine (no extrarenal elimination), an important corollary is that, in the steady state, the rate of generation can be assessed from the urinary excretion rate..
By substitution of eq. 9 and rearrangement, the plasma level can be related to the level of GFR and its non-GFR determinants (Gx, TRx, TSx, and Ex).
Px = ( Gx + TRx − TSx − Ex ) / GFR
Fig. 2.4 shows hypothetical changes in generation, excretion, balance, and plasma level of solute x after a 50% decrement in GFR, assuming TR, TS, and E are zero. In the steady state, changes in the plasma level would reflect reciprocal changes in GFR. For example, a decline in GFR to two-thirds, one-half, or one-third of the baseline level would be reflected by a rise in the plasma level to 1.5, 2.0, and 3.0 times the baseline level, respectively. Expression of the change in plasma level as its reciprocal (1/Px) would more clearly reflect the magnitude of changes over time in GFR in an individual.

Further rearrangement of eq. 10 provides the conceptual framework for estimating GFR from plasma solute levels of endogenous filtration markers.
GFR = ( Gx + TRx − TSx − Ex ) / Px
In practice, the non-GFR determinants of plasma solute levels are not measured. However, if the rates of these physiological processes were similar among all individuals and constant over time, then the level of GFR could be estimated directly from the inverse of the plasma concentration. Unfortunately, this is not the case for any of the currently used endogenous filtration markers.
Estimating Equations for Glomerular Filtration Rate
GFR estimating equations are equations that permit more accurate estimation of measured GFR from plasma levels of endogenous filtration markers and clinical and demographic variables than from the plasma level alone. GFR estimating equations are derived from regression analysis in which the level of measured GFR is related to the plasma solute concentration and observed clinical and demographic variables that serve as surrogates for the non-GFR determinants of plasma levels.
GFR = ( b × X + c × Y + d × Z ) / a × Px + ε
X, Y, and Z = numerical values for clinical and demographic variables
a, b, c, and d = coefficients relating Px and other variables to measured GFR
ε = the error based on uncertainty due to measurement, biological variability, and statistical techniques used to derive the coefficients
Estimating equations for GFR are often developed on the logarithmic scale, then exponentiated to report estimated GFR (eGFR) on the linear scale, and therefore have the appearance of:
eGFR = ( Px ) − a × X b × Y c × Z d × ε
In principle, use of multiple endogenous filtration markers with differing non-GFR determinants can improve the accuracy of GFR estimation. In multiple marker equations, the magnitude of the coefficient for each filtration would be closer to zero and the coefficients for clinical and demographic variables would be closer to 1.0, thereby lessening the dependence of eGFR on each filtration marker and factors associated with its non-GFR determinants.
Clinical use of GFR estimating equations would be facilitated if clinical laboratories reported eGFR when plasma concentrations of endogenous filtration markers were measured, and a single equation were used for each filtration marker or combination of markers. Here, we use the notation eGFRx to refer to estimated GFR based on plasma concentration of solute x. For endogenous filtration markers in current use, plasma and serum concentrations are generally equivalent.
Interpretation of Glomerular Filtration Rate Estimates
Development of accurate and generalizable estimating equations for widespread clinical use requires strict adherence to epidemiological and statistical principles. In general, it is recommended that equations be developed in a large study population (>500 subjects), including a variety of racial and ethnic groups for international comparisons, using high-quality GFR measurements; validated to have adequate precision and low bias against a gold standard measure of GFR in an independent study population; and practical to implement, taking into consideration cost, required data elements, assay considerations, and generalizability. Accuracy of GFR estimates in the validation population reflects bias, defined as average difference between the estimated and measured value for each subject, and precision, inversely related to the distribution of the differences between estimated and measured value for each subject. Table 2.3 lists some of the metrics that can be used for the assessment of bias, precision, and accuracy, as well as the causes for bias and imprecision. In general, knowledge of the sources of bias and imprecision can assist in interpretation of plasma levels of endogenous filtration markers and GFR estimates based on these levels. In this section, general principles are discussed; interpretation of GFR estimates from specific endogenous filtration markers are discussed separately later.
Criteria | Metric | Definition | Causes a |
---|---|---|---|
Bias | Median difference Median percent difference | Measured GFR-Estimated GFR b (Measured GFR-Estimated GFR)/Measured GFR | Systematic difference between development and validation population in GFR measurement error (clearance method or exogenous filtration marker) Assays for endogenous filtration markers Non-GFR determinants of endogenous filtration marker (selection criteria) Mean level of measured GFR |
Precision | SD difference IQR difference c IQR 96 difference c | Standard deviation of the differences Interquartile range of (Measured GFR−Estimated GFR) Interquartile range of ((Measured GFR−Estimated GFR)/Measured GFR) a 100 | Larger random variation in the validation than the development population True GFR (biological variation) GFR measurement error (clearance method or exogenous filtration marker) Non-GFR determinants of endogenous filtration markers |
Accuracy | Median absolute difference P30 RMSE | Median of the absolute value of eGFR−mGFR Percent of estimates within 30% of measured GFR Square root of mean (log Measured GFR−log Estimated GFR) | All of the above |
a Differences between development and validation population.
b May also be expressed as Estimated GFR–Measured GFR.
The coefficients for the clinical and demographic variables reflect average values for the relationship of the observed variables to the unmeasured surrogates in the development population. Systematic differences in these relationships between the development and validation populations is reflected as bias and generally reflects differences in selection between the development and validation populations. Random differences among individual patients are reflected as imprecision. In principle, use of multiple endogenous filtration markers with differing non-GFR determinants would cancel errors due to systematic bias in each filtration marker and improve precision.
There can be substantial variation among clinical laboratories in assays for endogenous filtration markers, leading to bias in GFR estimates between the development population and the validation population. This source of bias can be overcome by calibration of the clinical laboratory to the laboratory in which the equation was developed. In practice, this is best accomplished by standardization of clinical laboratories and reexpression of the GFR estimating equation for use with standardized values.
Measurement error in GFR in the development population is another source of inaccuracy in GFR estimates. This is a special case, however, because the difference is between measured and true GFR rather than between estimated and measured GFR. Systematic error in GFR measurement, due to the clearance method or the exogenous filtration marker, introduces a bias in GFR estimates compared with true GFR, which can lead to a bias in comparing GFR estimates to measured GFR in the validation population. Random error in GFR measurement leads to lower precision of GFR estimates compared with measured GFR than compared with true GFR in both the development and the validation populations.
A property of the statistical technique of regression is to “shrink” estimates to the mean of the development population. In the development population, the mean eGFR is unbiased, but higher values for measured GFR are systematically underestimated and lower values for measured GFR are systematically overestimated. In a validation population with a substantially different mean measured GFR, the estimates may be systematically biased due to “regression to the mean” of the development population. Because most GFR estimating equations are derived in a population with a wide range of GFR, this would lead to an underestimation of measured GFR in a validation population drawn from the general population, in which most subjects would be expected to have normal measured GFR, and an overestimation of measured GFR in a validation population with low GFR.
All of these factors tend to cause larger errors in GFR estimation at higher values, in large part because estimating equations are usually developed in study populations with a large number of patients with reduced GFR and because development on the logarithmic scale leads to larger errors at the higher levels when estimates are reexpressed on the linear scale. Thus eGFR is likely to be more accurate at lower values, as encountered in patients with kidney disease, and less accurate at higher values, as encountered in the general population.
Finally, it is difficult to estimate GFR in the nonsteady state because the change in the endogenous filtration marker lags behind the change in GFR (see Fig. 2.4 ). This limitation applies both to plasma levels of endogenous filtration markers and to GFR estimates based on plasma levels. Nonetheless, a change in the plasma levels and eGFR based on plasma levels in the nonsteady state can be a useful indication of the magnitude and direction of the change in kidney function. If the plasma level is rising due to declining kidney function, then the decline in eGFR would be less than the decline in measured GFR. Conversely, if the plasma level is falling due to rising kidney function, then the rise in eGFR would be greater than the rise in measured GFR. The more rapid the change in the filtration marker or in eGFR, the larger the change in measured GFR. As kidney function stabilizes, the endogenous filtration marker reaches a new steady state, and eGFR more accurately reflects measured GFR.
Endogenous Filtration Markers
Creatinine
Creatinine is the most commonly used endogenous filtration marker for estimation of GFR. Understanding basic concepts of metabolism, renal physiology, and analytical chemistry related to creatinine is essential to the interpretation of GFR estimates based on serum creatinine ( Table 2.4 ).
Creatinine | Cystatin C | Urea | |
---|---|---|---|
Overestimation of GFR (Lower Serum Levels) | |||
Generation | Reduction in muscle mass; vegetarian diet | Hypothyroidism, female sex, older age | Severe malnutrition and liver disease |
Renal handling | Proximal tubular injury; e.g., sickle cell disease | Not described | Decreased tubular reabsorption in absence of ADH |
Extrarenal elimination | Increase with severe reduction in GFR (gut) | Increased with severe reduction in GFR (spleen, diaphragm, heart, liver, and lungs) | Not described |
Assay | Not described | Not described | Not described |
Underestimation of GFR (Higher Serum Levels) | |||
---|---|---|---|
Generation | Higher muscle mass and ingestion of cooked meats and creatinine supplements | Corticosteroids, inflammation, hyperthyroidism, male sex, younger age, obesity, transplant recipients | Dietary protein intake, corticosteroids, diuretics, or tetracyclines; absorption of blood from the gut, infection, acute kidney injury, trauma, congestive heart failure, and sodium depletion |
Renal handling | Decreased secretion with drugs such as cimetidine, trimethoprim, possibly fenofibrate | Not described | Increased tubular reabsorption in presence of ADH |
Extrarenal elimination | Decrease with antibiotic use | Not described | Not described |
Assay | Keto acids, some cephalosporins may interfere with alkaline picrate; flucytosine may interfere with enzymatic by as much as 60% | At high levels of bilirubin interference with the PETIA assay | Ammonium in reagents or use of ammonium heparin; drugs such as chloral hydrate, chlorbutanol, and guanethidine |
1
Structure and Function
Creatinine is a 113-dalton amino acid derivative that serves as a nitrogenous waste. It is distributed throughout total body water and has no known toxicity.
2
Plasma Levels
The normal level of GFR is sufficient to maintain a low concentration of creatinine in serum, approximately 0.64 to 1.36 mg/dL.
3
Generation
Creatinine is generated in muscle from the nonenzymatic conversion of creatine and phosphocreatine. Creatine is synthesized from arginine and glycine in the liver and actively concentrated in muscle. Thus creatinine generation reflects the size of the creatine pool, which is proportional to muscle mass. In the steady state, creatinine generation can be estimated by creatinine excretion, and related to age, gender, and body size.
Ucr × V = 28 . 2 − 0 . 172 × age ( men )
Ucr × V = 21 . 9 − 0 . 115 × age ( women )
These equations do not take into account racial and ethnic differences in muscle mass. African American (black) males and females have higher muscle mass and consequently higher creatinine excretion than their Caucasian (white) counterparts. Asians have lower muscle mass and lower creatinine excretion rate than their Caucasian counterparts. Other equations to estimate creatinine excretion have been proposed, but precision is limited; approximately 20% of estimates deviate by more than 30% of measured creatinine excretion rate.
Creatinine generation is affected by diet and disorders of skeletal muscle. Muscle wasting is associated with a decreased creatine pool, leading to decreased creatinine generation and excretion. Reduction in dietary protein causes a decrease in the creatine pool by 5% to 15%, probably by reducing the availability of creatine precursors. Of greater importance is the effect of creatine in the diet. Creatine is contained largely in meat; elimination of creatine from the diet decreases urinary creatinine excretion by as much as 30%. Conversely, ingesting a creatine supplement increases the size of the creatine pool and increases creatinine excretion. Meat intake also affects creatinine generation and excretion independent of its effect on the creatine pool. During cooking, a variable amount of the creatine in meat is converted to creatinine, which is absorbed from the gastrointestinal tract. After ingestion of cooked meat, there is a sudden transient increase in the serum creatinine concentration and urinary creatinine excretion.
4
Renal Handling
- a.
Glomerular filtration. The small molecular diameter of 0.3 nm and the lack of binding to plasma proteins assures the free passage of creatinine through the glomerular capillary wall into the Bowman space (sieving coefficient of 1.0).
- b.
Tubular secretion. Creatinine is actively secreted by the tubules, probably by the same pathway used for other organic cations in the proximal tubule; hence creatinine clearance exceeds GFR.
Ucr × V = GFR × Pcr + TScr
Ccr = GFR + TScr / Pcr
- c.
Tubular reabsorption. To a limited extent, creatinine may also be reabsorbed by the tubules, possibly due to its passive back-diffusion from the lumen to blood because of the high tubular creatinine concentration that occurs during low urine flow. Based on the clearance ratios observed in these studies, the maximum effect of creatinine reabsorption probably would be a 5% to 10% decrease in creatinine clearance.
5
Extrarenal Elimination
Extrarenal loss of creatinine is not detectable in normal individuals but may account for a larger proportion of daily creatinine generation in patients with severe decrease in GFR. Thus in patients with kidney disease, creatinine excretion underestimates creatinine generation:
Ucr × V = Gcr − Ecr
6
Assay
Creatinine can be measured easily in serum, plasma, and urine by a variety of methods. No systematic differences between serum and plasma have been noted. The gold standard method for creatinine assay is isotope dilution mass spectrometry (IDMS) using either gas or liquid chromatography. Various methods are in use in clinical laboratories to assay serum and urine creatinine. Calibration of autoanalyzers differs among clinical laboratories, irrespective of the method for measurement of serum creatinine. The National Kidney Disease Education Program (NKDEP) and the International Federation of Clinical Chemistry and Laboratory Medicine (IFCC) have standardized serum creatinine assays to methods traceable to IDMS to minimize differences in across clinical laboratories. However, standardization does not eliminate patient-specific factors that interfere with creatinine measurement, as discussed below.
- a.
Alkaline-picrate methods. The classic method used the Jaffe reaction, in which creatinine reacts directly with picrate ion under alkaline conditions to form a red-orange complex that is easily detected and quantified. In normal subjects, up to 20% of the color reaction in serum or plasma is due to solutes other than creatinine, resulting in an apparent creatinine value that is 20% higher than the true value. Noncreatinine chromogens are not present in sufficient concentration in urine to interfere with creatinine measurement. In patients with kidney disease, noncreatinine chromogens are not retained to the same degree as creatinine; consequently, the overestimation of serum creatinine is reduced, as is the underestimation of creatinine clearance at lower GFR. The kinetic alkaline-picrate method takes advantage of the differential rate of color development for noncreatinine chromogens compared with creatinine. It significantly reduces but does not eliminate the positive interferences described previously. As discussed earlier, with standardization of creatinine assays, the discrepancy between creatinine clearance and GFR in normal individuals became apparent.
- b.
Enzymatic methods. To circumvent interferences in the alkaline picrate reaction, a variety of enzymatic methods have been developed. Two are in use in clinical laboratories: the creatinine iminohydrolase method and the creatininase, creatinase, and sarcosine oxidase method. Both methods have been reported to have fewer interferences than the alkaline-picrate methods.
- c.
HPLC. HPLC is a fairly sensitive and analytically specific method for measuring serum creatinine. Many of the protocols include deproteinization to obviate the effects from interfering compounds. All of the commonly used methods are imprecise in the lower range of serum creatinine. The imprecision makes it difficult to interpret changes in serum creatinine within the normal range, as one cannot readily distinguish between differences in serum creatinine levels due to errors in the assay or due to biological variability in GFR.
7
Creatinine as a Filtration Marker
Based on the previous considerations, the relationship of GFR to serum creatinine is expressed as follows:
GFR = ( Gcr − TScr − Ecr ) / Scr
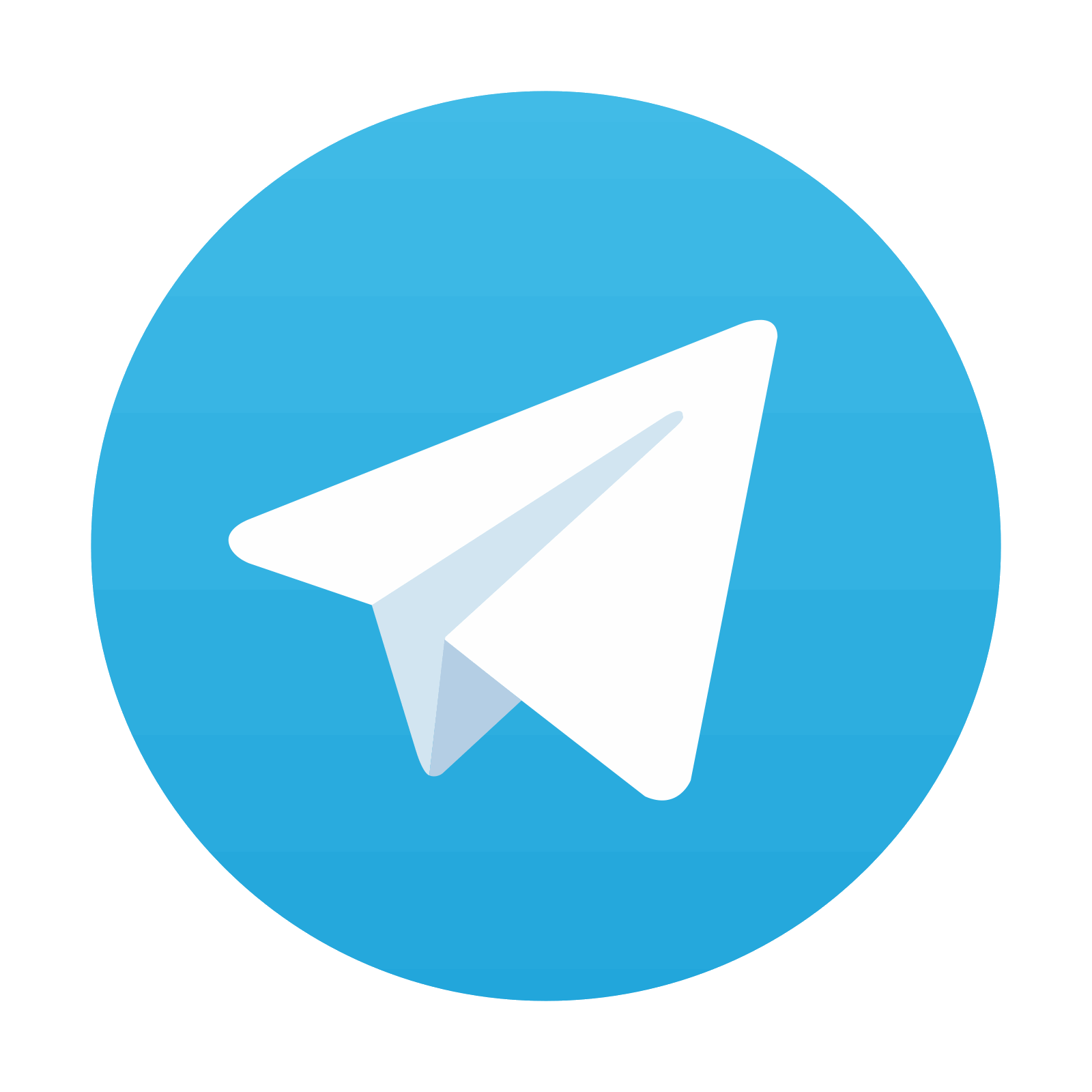
Stay updated, free articles. Join our Telegram channel
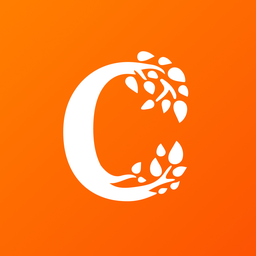
Full access? Get Clinical Tree
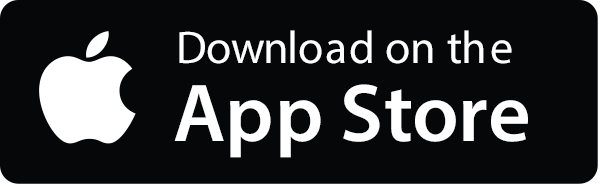
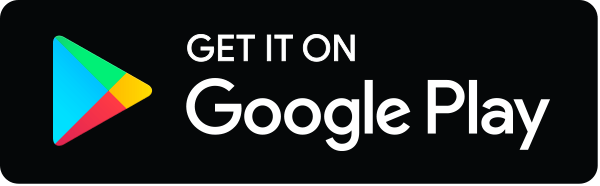