6. LIVER AND BILIARY SYSTEM
After studying this chapter you should be able to:
1. Understand the role of the liver in the digestive process and the excretion of waste metabolites and toxic substances.
2. Understand the relationship between the structure of the hepatobiliary tract and its function in the secretion and storage of bile.
3. Understand the mechanisms of secretion of the important components of bile, and their recycling via the enterohepatic circulation.
4. Understand the mechanisms of control of bile secretion and its release into the duodenum.
Introduction
The numerous functions of the liver can be divided into two broad categories:
1. Those concerned with the processing of absorbed materials and synthetic reactions
2. Those concerned with secretion and excretion.
This chapter is concerned with the secretory and excretory roles of the liver. The processing of absorbed nutrients and the role of the liver in the control of energy metabolism are discussed in Chapter 9.
The most important exocrine functions of the liver are:
• The provision of bile acids and alkaline fluid for the digestion and absorption of fats, and for the neutralization of gastric acid in the intestines
• The degradation and conjugation of waste products of metabolism
• The detoxification of poisonous substances
• The excretion of waste metabolites and detoxified substances in bile
• Detoxified substances and waste metabolites are eliminated from the body either in the bile, via the gastrointestinal tract, or via secretion from the liver into the blood for subsequent excretion by the kidneys.
The liver has enormous reserves of function, and normal homeostasis can be maintained even after three quarters of it have been removed. Clinical manifestation of liver disease therefore implies considerable damage to the organ.
Gallstones form in the gall bladder and biliary tract as a consequence of various derangements of the hepatobiliary system. The problems encountered in a patient with gallstone disease are given in this chapter to illustrate many of the roles of the liver in secretion and excretion. A second case history involving a patient who had taken an overdose of paracetamol is used to emphasize the important role of the liver in the detoxification of drugs.
Overview of the functioning of the hepatobiliary system
The anatomical arrangement of the liver, gall bladder and biliary tract is shown in Figure 6.1. The liver is continually secreting substances both into the blood, and into the bile. Bile is both a secretory fluid and an excretory medium. In the human, it is stored between meals in the gall bladder, where it is concentrated. During a meal it is released from the gall bladder and enters the cystic duct that in turn drains it into the common bile duct. The bile enters the small intestine at the level of the duodenum. Its entry into the small intestine is controlled by a smooth muscle sphincter: the sphincter of Oddi.
The gall bladder is surrounded by smooth muscle. Between meals, when the gall bladder smooth muscle is relaxed, the sphincter of Oddi is closed, preventing the bile from entering the small intestine. Consequently, the bile passes into the gall bladder where it is stored and concentrated. Contraction of the gall bladder forces the bile into the common bile duct. At the same time the smooth muscle in the sphincter of Oddi relaxes, and the sphincter opens to allow the bile to enter the duodenum. Food in the duodenum is the main stimulus for gall bladder contraction.
Anatomy and morphology of the liver
The liver is the largest single organ in the body. In the adult it comprises approximately one-fiftieth of the body weight. In the infant it is proportionately even larger. It consists of right and left lobes (Fig. 6.2A), the right lobe being six times the size of the left in the adult.
The liver is composed of lobules (Fig. 6.2B). In the centre of each lobule is the central canal, in which lies a hepatic vein, which is a tributary of the inferior vena cava. Columns of liver cells (hepatocytes) and sinusoids radiate out from the central canal. Several portal tracts lie at the periphery of each lobule. Each tract (or ‘portal triad’) contains a bile duct, a branch of the portal vein, and a branch of the hepatic artery.
The liver has a double blood supply: the hepatic artery supplies the liver with oxygenated blood from the lungs, and the portal vein supplies it with nutrient-rich blood from the intestines (see Ch. 1). The arterial blood comprises approximately 20% of the total blood supply of the liver and the portal venous blood, approximately 80%. The arterial blood and the portal venous blood mix together in the liver sinusoids. The sinusoidal blood drains away via the hepatic veins to the vena cava. This direction of flow is determined by the relatively higher pressure of the blood in the portal vein compared to the central vein.
In liver cirrhosis, scar tissue replaces normal healthy tissue. This scar tissue distorts the normal structure and regrowth of liver cells and blocks the flow of blood through the organ. This disease is described in Box 6.1.
Box 6.1
In cirrhosis of the liver scar tissue replaces normal healthy tissue and blocks the flow of blood from the portal vein through the organ. This leads to reduced synthesis of proteins and other molecules by the liver and reduced oxidative capacity. Metabolism of bile constituents, and drug detoxification, and secretion and excretion of bile constituents become inadequate to maintain health.
Liver cirrhosis has many causes, including:
• Chronic alcoholism. Cirrhosis does not usually develop until after more than 10 years of alcohol abuse. This is a major cause of liver cirrhosis in the western world
• Chronic hepatitis C, B or D. Hepatitis C virus causes low-grade damage, which over the course of many years can lead to cirrhosis. This is a major cause of liver cirrhosis. It was commonly transmitted by blood transfusion before routine testing for hepatitis C virus was available. Hepatitis B virus is the most common cause of liver cirrhosis in the third world but is less common in more developed countries
• Autoimmune disease: the immune system attacks the liver causing inflammation and tissue damage which can eventually lead to cirrhosis
• Inherited diseases including α1-antitrypsin deficiency, haemachromatosis, Wilson’s disease, galactosaemia and glycogen storage diseases
• Drugs, toxins and infections. Severe reactions to prescription drugs, prolonged exposure to environmental toxins, parasitic infection (with schistosomes) can also cause liver cirrhosis.
The signs and symptoms of liver cirrhosis include fatigue, weight loss, nausea, abdominal pain, spider-like blood vessels on the skin, oedema of the legs and abdomen (ascites), jaundice (see Box 6.2), gallstones (see Case 6.1, Case 6.1, Case 6.1, Case 6.1, Case 6.1 and Case 6.1), itching (due to bilirubin being deposited in the skin) and a tendency to bleed easily (due to reduced clotting factor synthesis in the liver).
The treatment of liver cirrhosis depends on the cause of the condition and the complications experienced. The damage cannot be reversed but the progression of the disease can be arrested or delayed by, for example cessation of alcohol abuse or medication to treat infections and other causes. When liver damage is so pronounced that the liver stops functioning, a transplant is necessary. The survival rate after liver transplantation is over 90% now that effective immune-suppression drugs are available.
The liver is covered by a fibroconnective tissue capsule known as the capsule of Glisson, from which thin connective tissue septa enter the organ to divide it into lobes and lobules. The capsule is covered by peritoneum, except in an area known as the ‘bare area’ which is in direct contact with the diaphragm.
The secretory system of the liver begins with minute tubules, the canaliculi. These are formed by oppositely aligned grooves in the contact surfaces of adjacent hepatocytes (Fig. 6.2 and Fig. 6.3). The membrane of each liver cell contributes to several bile canaliculi. Bile secreted into the canaliculi flows in the opposite direction to the flow of blood in the sinusoids. The canaliculi drain into terminal bile ductules. The ductules converge to form intralobular ducts, and these converge to form interlobular ducts, which in turn converge to form the right and left hepatic bile ducts. These converge outside the liver to form the common hepatic bile duct.
Histology
The major type of cell in the liver is the hepatocyte that is an epithelial parenchymal cell. The hepatocytes are arranged in plates which branch and anastomize to form a three-dimensional lattice (Fig. 6.2). Between the plates are the blood-filled sinusoids. In this respect the liver resembles an endocrine gland. There is usually only one layer of hepatocytes between the sinusoids.
The sinusoidal spaces differ from blood capillaries in that they are of greater diameter and their lining cells are not typically endothelial. The basal lamina around the sinusoids is incomplete and this enables direct access of the plasma to the surface of the hepatocyte. This allows active metabolic exchange between the blood and the cells (Fig. 6.2C). The perisinusoidal space is an interstitial space that contains reticular and collagenous fibres. A few mesenchymal cells called lipocytes produce the fibres. Two main cell types are present in the sinusoidal lining. These are endothelial cells and Kupffer cells. They lie in a mesh of fine reticular fibres. The endothelial cell has small elongated nuclei and greatly attenuated cytoplasm. The cytoplasm may interdigitate with cytoplasmic processes from adjacent cells of the same type or another type. They contain few organelles but numerous pinocytotic vesicles. They also contain large fenestrae that are not closed by a diaphragm. Kupffer cells are phagocytic and often contain degenerating red cells, pigment granules, and iron-containing granules. They have large nuclei and extensive cytoplasm, with processes that extend into, and sometimes across, the sinusoidal space. They increase in number when required for phagocytosis, possibly by differentiation of the endothelial type of cell.
Hepatocytes
The hepatocyte is a polygonal cell with a clearly defined cell membrane, which is closely apposed to the cell membranes of adjacent hepatocytes (Fig. 6.2 and Fig. 6.3). The membranes of adjacent cells are partially separated to form a bile canaliculus. The plasmalemma of adjacent hepatocytes shows irregularities with tight junctions, spot desmosomes and gap junctions. These separate the canaliculus from the rest of the intercellular space (Fig. 6.2C).
The plasma membrane of hepatocytes is specialized in certain regions. Adjacent to a sinusoidal blood space the hepatocyte is separated from the wall of the sinusoid by the perisinusoidal space (the space of Disse) and at this location the plasma membrane of the hepatocyte has numerous long microvilli. Vesicles and vacuoles are present in the subadjacent cytoplasm (Fig. 6.2C). The microvilli provide a large surface area for absorption and secretion.
The nuclei in different hepatocytes show considerable variation in shape and size and in some cases the cells are binucleate. Clumps of basophilic material are present in all cells. There are numerous small mitochondria throughout the cytoplasm of the hepatocyte. The structure of all hepatocytes is broadly similar but the cytoplasm of the cells shows a gradual variation with the distance of the cell from the periphery. The differences are related to the differences in functional activity of the peripherally and centrally positioned cells. The hepatocytes closest to the afferent blood supply, the ‘periportal’ cells, are exposed to the highest concentrations of nutrients and oxygen and those in the central region, the ‘perivenous’ cells, near to the efferent outflow, are exposed to the lowest concentrations. The periportal cells are the most active in the uptake from the blood of bile salts and in the secretion of many bile constituents into the canaliculi as well as in oxidative metabolism and gluconeogenesis (Fig. 6.4). After feeding, glycogen is deposited first in the periportal cells. It is only after a heavy carbohydrate meal that the more centrally located perivenous cells store glycogen. Moreover, when the blood sugar concentration falls, glycogen is removed first from the perivenous cells. The perivenous cells, which are exposed to depleted plasma, are the more active in biotransformation reactions and the secretion of potentially toxic xenobiotic and endobiotic substances. They are also more active in glycolytic and ketogenic reactions. Under certain conditions fat is deposited in the hepatocytes and it appears first in the more centrally disposed cells. Thus the cytosol of a given hepatocyte exhibits differences in composition at different times in relation to feeding and whether fat or glycogen has been deposited.
The canaliculus
The lumen of the canaliculus is approximately 0.75μm in diameter. Microvilli project from the canalicular membrane into the lumen, providing a large surface area for secretion. Membranes of adjacent hepatocytes are joined by tight junctions near the canaliculus (Fig. 6.2C). These junctions are leaky and permit paracellular exchange between the plasma and the canaliculus.
The canaliculus is involved in transport of substances into the lumen, but it is also a contractile structure. Actin filaments are present in the microvilli, and both actin and myosin fibres are present in the cytoplasm surrounding the canaliculus. Contractions of the canaliculi can be stimulated by extracellular ATP. The contractions involve actin–myosin interaction as in smooth muscle cells. They probably pump bile towards the ducts. Atony (lack of contractile function) of the canaliculus causes cholestasis (reduced bile flow).
The junctions of the bile canaliculus with the bile ducts at the periphery of a lobule consist of an intermediate structure called the ductules or canals of Hering. Here the hepatocytes that form the canaliculus are gradually replaced by smaller cells with dark nuclei and poorly- developed organelles. These are the ductule cells. They are underlain by a distinct basal lamina. The lumen of the ductule eventually joins that of a bile duct in the portal area.
Extrahepatic ducts
The extrahepatic ducts are lined by tall columnar epithelium (Fig. 6.5) that secretes mucus. There is a layer of connective tissue beneath the epithelium, with numerous elastic fibres, mucous glands, blood vessels and nerves. In the common bile duct there is also a layer of smooth muscle cells. These cells are sparse in the upper region of the duct but form a thicker layer of oblique and transverse fibres in the regions of the sphincter of Oddi near the duodenum (see below).
Bile
Composition and functions
Bile is secreted at a rate of 250–1000mL/day in the adult. It is isosmotic with blood plasma. It is a composite of two different secretions; one originating in the hepatocytes, and the other in the cells that line the bile ducts (Fig. 6.5). The two secretions mix together in the ducts.
Secretion of the duct cells
The secretion from the duct cells is a watery alkaline fluid that is rich in bicarbonate. It comprises approximately 25% of the total bile volume. Its function is, first, to provide an appropriate pH for the process of micelle formation (see below), which requires a neutral or slightly alkaline environment. Second, it contributes (together with pancreatic juice and intestinal secretions) to the neutralization of stomach acid in the intestinal chyme. This is important both for micelle formation and digestive enzyme action in the small intestine (see Ch. 8). In addition the neutralization of acid in the duodenum protects the mucosa from ulceration. The secretion contains Na+, K+, Cl− and HCO3− ions. Its composition is similar to that of alkaline pancreatic juice. At basal rates of secretion the ionic composition resembles that of plasma. However as the flow increases upon stimulation (by a meal) the Cl− concentration decreases and the HCO3− concentration increases. This is due to the presence of a Cl−/HCO3− exchange mechanism in the duct cells. HCO3− ions are extracted from the bile and Cl− is added to it, a process similar to that involved in the secretion of alkaline juice from the pancreatic duct cells (see Ch. 5). At high flow rates, the bile is not in contact with the duct cells for sufficient time to allow appreciable modification to take place and there is proportionately less bicarbonate extracted, resulting in a more alkaline bile. Thus, the secretion becomes more alkaline at flow rates higher than the basal level.
The volume of the alkaline secretion, unlike the secretion produced by the hepatocytes, is not directly determined by the concentration of bile salts in the blood. It has been termed the ‘bile acid-independent’ component of bile. The control of this secretion during a meal, like that of alkaline pancreatic juice and the alkaline fluid secreted from Brunner’s glands in the duodenum, is via the release into the blood of the hormone secretin, from the walls of the duodenum. This occurs mainly in response to the presence of acid in the duodenum. The hormone circulates to stimulate all of these alkaline secretions. As it is released in response to acid chyme in the duodenum this mechanism provides a feedback control of the pH of the intestinal contents.
Secretion from the hepatocytes
The hepatocytes secrete a primary juice into the canaliculi. It contains a number of inorganic monovalent and divalent ions, and various organic substances (Table 6.1). The latter include lipids; bile acids, lecithin and cholesterol, all of which are sequestered together in micelles. Bile secretion is a major route whereby cholesterol is lost from the body. The bile acids are essential for the effective digestion and absorption of dietary fats. There are also some proteins in bile, including albumin, polymeric immunoglobulin A (pIgA) that protects the biliary tract and the upper intestines from infection, and some plasma-derived enzymes. Bile also contains bile pigments, chiefly bilirubin, that have been conjugated with glucuronic acid. The pigments are breakdown products of haemoglobin. Bile also contains numerous other compounds that are extracted from the blood, metabolized by the liver, and excreted. Many of these substances are potentially toxic endogenous or exogenous substances such as steroid hormones, drugs and environmental toxins that have been detoxified and conjugated in the liver. Conjugation serves to increase the polarity of a substance and therefore its solubility in water (see below). Bile remains isosmotic with plasma at different rates of flow. This implies that an increase in secretion of bile acids and metabolites by the liver is accompanied by an increase in water secretion resulting in an increase in bile volume. This is known as the choleretic effect.
Loss of gall bladder bile via a fistula can quickly deplete K+ reserves, unless replacement therapy is started. | ||
Constituent electrolytes | Hepatic bile (mM) | Gall bladder bile (mM) |
---|---|---|
HCO3− | 28 | 10 |
Cl− | 100 | 25 |
K+ | 5 | 12 |
Na+ | 145 | 130 |
Ca2+ | 5 | 23 |
Organic molecules | ||
Bilirubin | 0.7 | 5.1 |
Cholesterol | 2.6 | 16.0 |
Lecithin | 0.5 | 3.9 |
Bile salts | 26.0 | 145.0 |
Biliary lipids
The structures of the major lipids present in bile are shown in Figure 6.6. The bile acids are derivatives of cholest-erol and contain the cyclopentanoperhydrophenanthrene nucleus. One, two or three alcohol groups are attached to this nucleus and there is a short hydrocarbon chain ending in a carboxyl group (Fig. 6.6). Primary bile acids (cholic acid and chenodeoxycholic acid) are synthesized by the liver hepatocytes. Secondary bile acids (deoxycholic acid and lithocholic acid) are formed by dehydroxylation of primary bile acids in the intestines, by bacteria (Fig. 6.6). The bile acids are usually conjugated in the hepatocyte, with amino acids, largely glycine or taurine. The ratio of glycocholates to taurocholates is normally approximately 3:1 but the exact proportions depend on the relative availability of the two amino acids. The conjugated primary and secondary bile acids are reabsorbed actively in the ileum (see Ch. 8). However, bile acids may be deconjugated by bacteria in the small intestine and colon. Some of the unconjugated bile acids are absorbed by passive diffusion. Bile acids synthesized de novo in the liver, and absorbed unconjugated bile acids, are reconjugated in the liver. In physiological fluids bile acids form salts with Na+ and K+ ions.
Uptake of bile salts from the blood into the hepatocyte is an active process that occurs against a concentration gradient (Fig. 6.7). It derives its energy from a Na+/K+-ATPase that pumps Na+ out of the cell and involves a Na+/bile salt cotransporter system located in the sinusoidal membrane. The process is driven by the electrochemical gradient for Na+ set up by the pumping out of Na+ ions. Another mechanism for bile acid transport, which involves a transporter with a wider specificity, has also been characterized. The different bile salts compete with each other indicating that they share the same transporter. Inside the hepatocyte the bile salts bind to protein, thereby keeping the intracellular concentration of free bile salts low. These proteins may be involved in transport of the bile acids through the cell.
Bile salts are secreted into the canaliculus against a considerable electrochemical gradient. The transport across the canalicular membrane is Na+-independent. The energy may be partly derived from the membrane potential which is approximately 40mV (negative inside the cell), but an ATPase-dependent pump that is specific for bile acids is present in the canalicular membrane and this is the major mechanism for bile salt transport across this membrane (see Fig. 6.7). It is distinct from the Na+ gradient-driven bile acid uptake transporter in the sinusoidal membrane.
Bile acids are held in micelles in bile. They can be concentrated several-fold in hepatocyte bile as they are still held in micellar form. Bile acids are powerful detergents, and their sequestration in micelles may reduce their detergent and cytotoxic actions.
The major phospholipid in bile is a phosphatidylcholine (lecithin) with a unique fatty acid pattern: palmitic acid forms the outer ester bond and either oleic acid or linoleic acid the inner ester bond (Fig. 6.6). The immediate source of this phospholipid is a preformed pool in the liver cell membranes. The cholesterol in bile is also mainly derived from a preformed pool but an appreciable proportion is derived via de novo synthesis in the liver. The cholesterol in bile is not esterified to any significant extent (Fig. 6.6).
Inside the hepatocytes the phospholipid and cholesterol probably exist as components of membranes of intracellular vesicles. The membranes of these vesicles are incorporated into the plasma membrane by fusing with it. The rate of secretion of phospholipid and cholesterol appears to be linearly related to the rate of bile salt secretion. Bile salts are secreted into the canaliculus first. The detergent action of these may be responsible for removing the other lipids from the canalicular membrane. The ratio of cholesterol to phospholipid is fairly constant (approx. 0.3 in the human). Some biliary phospholipid and cholesterol is present in bile in vesicles. These vesicles can incorporate bile salts and are gradually converted to micelles.
Micelle formation
Bile salts are essential for the formation of micelles in bile. The bile salt molecule is amphiphilic; the roughly planar ring system is hydrophobic and forms one side of the molecule. The alcohol groups, the carboxyl group, and the peptide bond of the bile acid, all project from the other side, imparting a net negative charge, and therefore a hydrophilic nature to that side of the molecule (Fig. 6.8). A micelle has a hydrophilic shell region and a hydrophobic core region. Newly formed (primary) micelles are initially composed of bile salt molecules. The bile salts orientate themselves in the micelle with the hydrophobic side in the core and the hydrophilic side in the shell (Fig. 6.8). Primary micelles can sequester very little cholesterol. However, they take up phospholipid to form mixed micelles. Phosphatidylcholine is also an amphiphilic molecule; the long chain fatty acyl chains forming the hydrophobic domain that resides in the core region of the micelle and the phosphorylcholine group the hydrophilic domain which projects into the shell region (Fig. 6.8). The mixed micelle can hold more cholesterol than the primary micelle. In the presence of phosphatidylcholine larger micelles tend to form than in its absence. It is therefore known as a ‘swelling’ amphiphile. Cholesterol, which is extremely insoluble in water, resides in the core of the micelle. As the net charge on all micelles is negative they repel each other, thereby preventing coalescence, and inducing the formation of a stable suspension. The negatively charged micelle collects an outer shell of cations, mainly Na+ ions. A micelle is disc-shaped, and its thickness approximates that of a lipid bilayer.
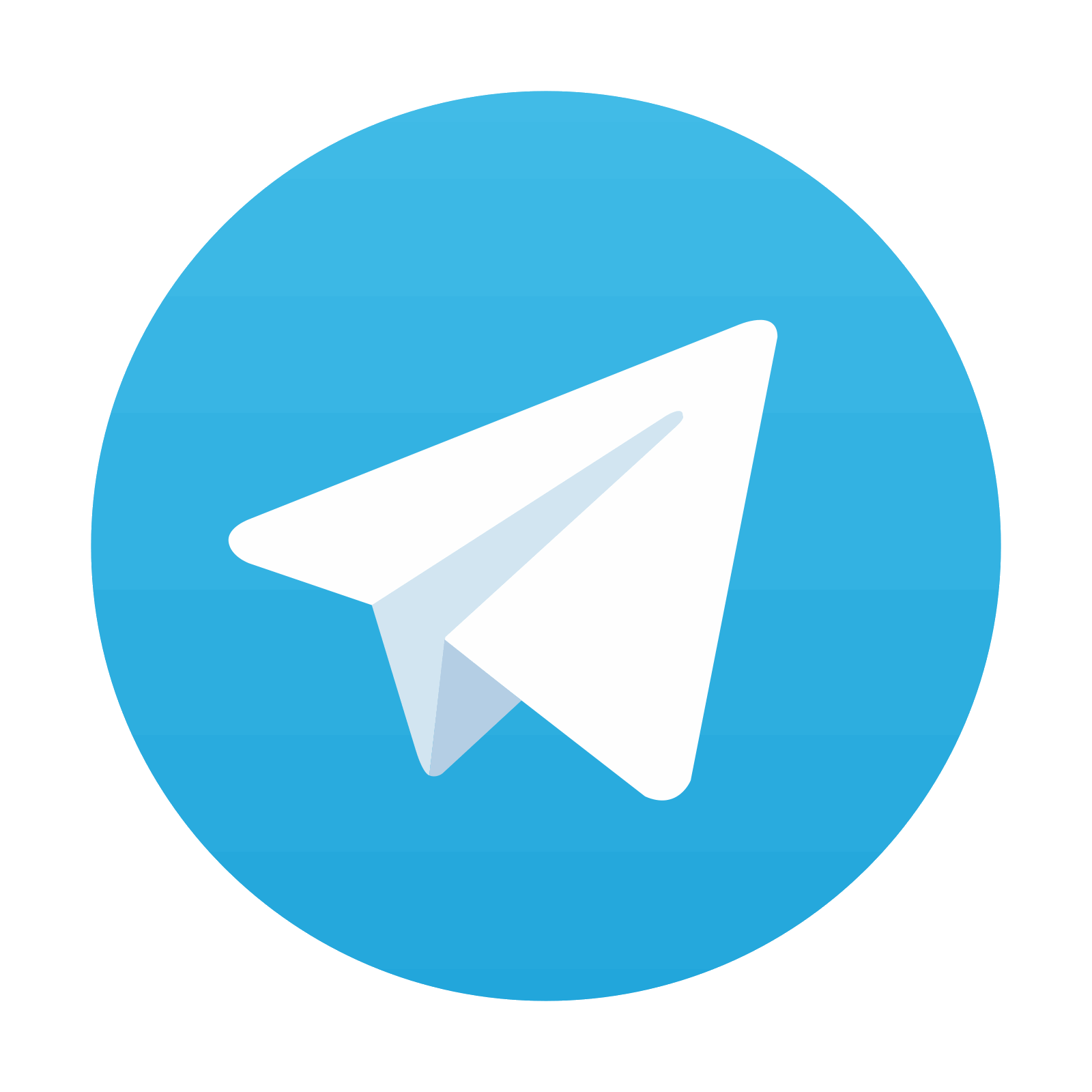
Stay updated, free articles. Join our Telegram channel
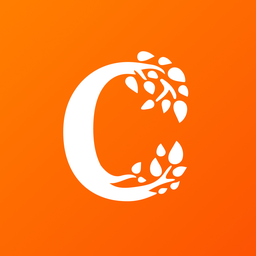
Full access? Get Clinical Tree
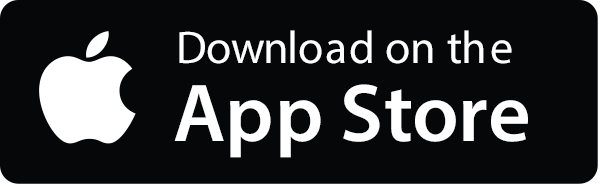
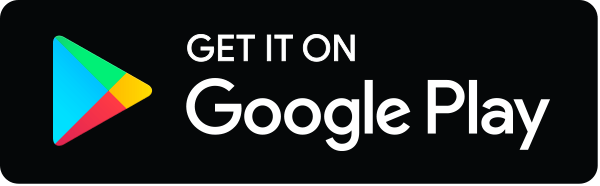