59 Geert G. Tailly Department of Urology and Pediatric Urology, AZ Klina, Kapellen, Belgium Prior to the introduction of extracorporeal shock‐wave lithotripsy (ESWL) and other minimally invasive techniques such as percutaneous nephrolithotomy and ureterorenoscopy, open surgery was the principal treatment modality for urolithiasis. The first extracorporeal shock‐wave lithotripsy (ESWL) that was performed on a stone‐bearing patient in 1980 therefore thoroughly revolutionized modern stone management. As early as 1963 physicists at Dornier, an aircraft manufacturer in Friedrichshafen, Germany, had investigated the impact of raindrops on flying objects, as these impacts caused shock waves which not only damaged the outer shell of airplanes but also structures within. During these experiments an engineer at Dornier accidentally discovered the effects of these shock waves on biological tissue. At the same time the German Ministry of Defense was also interested in the interaction between shock waves and biological tissue. In 1974 a partnership to conduct research on the application of shock waves in humans was formed between Dornier Development and Research (W. Hepp and G. Hoff), the Department of Urology at the Ludwig‐Maximilians Universität in Munich (E. Schmiedt and F. Eisenberger), and the Institute of Surgical Research at the same university (W. Brendel and Ch. Chaussy). Funding was obtained through the German Research and Technology Ministry [1–3]. Early on it was found that shock waves caused no damage when traveling through muscle, fat, or connective tissue, except at transition zones with high acoustic impedance. In the course of this project someone then formed the idea to fragment kidney stones in the human body [1, 2]. After extensive experiments the first lithotriptor for human treatment, the Dornier Human Model 1 or HM1, was built, in 1979. Patient selection for the first treatments was very strict: The first patient was successfully treated using a Dornier HM1 on 7 February 1980 by Christian Chaussy, B. Forssmann, and D. Jocham [4]. The results of the first clinical study on 221 treatments in 206 patients were published in 1982 [5]. In order to promote the new technique as reproducible and safe, the HM1 needed several improvements: The Dornier HM3 was born. In 1983 the second lithotripsy center was opened in the Department of Urology (F. Eisenberger) of the Katharinen Hospital in Stuttgart. In March 1984 the first Dornier HM3 in the United States was installed in the Methodist Hospital in Indianapolis (Daniel M. Newman and James E. Lingeman). A study for marketing approval by the US Food and Drug Administration was monitored by Georges Drach [6]. The rest is history. Originally lithotripters were devices only dedicated to shock‐wave lithotripsy (SWL). With modern stone management demanding a judicious combination of endourologic techniques and SWL, there was an evolution toward the construction of multifunctional urologic workstations. A modern stone center needs to tailor the most appropriate treatment to both the patient and their stone. A multifunctional workstation where both endourological techniques and ESWL can be performed in great comfort therefore seems an essential tool. The Dornier HM3 lithotripter represented the archetype of the first‐generation lithotripter, characterized by a large water bath in which the patient was immersed for optimal shock‐wave coupling. Equipped with an electrohydraulic shock‐wave source and an ellipsoidal reflector with a small aperture to focus the shock wave, the treatment required general or spinal anesthesia. A bidirectional fluoroscopic imaging system allowed targeting to move the stone into the therapeutic focus F2. Multifunctional or multidisciplinary use was impossible. The results achieved with this device are still considered the basis for comparison in evaluating all new devices. Second‐generation lithotripters utilize an electrohydraulic, electromagnetic, or piezoelectric shock‐wave source. Transmission of the shock wave is provided by means of a water cushion or partial water bath. Stone localization is performed with an ultrasonic or fluoroscopic imaging system [7–10]. The anesthesia requirements have been reduced so that SWL can be performed without any medication or only in analgosedation. Multifunctional or multidisciplinary use is limited. Third‐generation lithotripters are also equipped with an electrohydraulic, electromagnetic, or piezoelectric shock‐wave source. All systems allow anesthesia‐free treatments. Apart from that they use both fluoroscopy and ultrasound, alternately or simultaneously, to target the stones. Finally, all components are integrated into a multifunctional system with a treatment table allowing both SWL and endourologic procedures to be performed on the same device. Shock waves are acoustic waves. Acoustic waves are mechanical waves consisting of pressure and density variations which can travel through media in any of its phases: gaseous, liquid, or solid. They are also referred to as pressure waves. Acoustic waves propagate through a medium by alternating decompression and compression of the medium. At the interfaces of the media they pass, absorption, reflection, or refraction of the shock waves can occur [11]. An acoustic wave of very short duration is called an acoustic pulse and a shock wave is a very short acoustic pulse. On the wave front the positive pressure rises in a very short time from the ambient pressure to the maximum pressure. Afterwards a short phase of underpressure follows. While the wave propagates through the medium, it gets continuously steeper until it forms a shock wave. The generated shock wave is characterized by a sudden drop from overpressure to underpressure. The short phase of underpressure generates cavitation in the transmission medium. The bubbles induced by cavitation subsequently collapse violently and create microjets or secondary shock waves. For medical purposes shock waves are generated in water and transmitted into the patient’s body using a (partial) water bath or a water cushion for coupling. Water is used as transmission medium due to having comparable acoustical properties to human tissue, leading to impedance matching with low reflection at the contact surface between the water bath or water cushion and the patient. In the Dornier HM3, the first lithotripter commercially available, shock waves were generated using an electrohydraulic shock‐wave source. In second‐ and third‐generation lithotripters any of three principles of shock‐wave generation are used: electrohydraulic, electromagnetic, or piezoelectric. Electrohydraulic shock‐wave generation is based on an underwater spark discharge. A spark plug with two opposing electrode tips is positioned in the focus F1 of an ellipsoidal reflector. A capacitor of about 100 nF connected to the spark gap is charged to a voltage of 15–30 kV and then abruptly discharged, producing a fast‐growing plasma channel between the electrode tips and resulting in rapid vaporization of the surrounding water like a micro‐explosion. This releases a spherical shock wave that is reflected by the wall of the ellipsoidal reflector towards the therapeutic focus F2 of the reflector. The intensity of the shock wave can be adjusted by changing the discharge voltage. The treatment depth and focusing are defined by the geometrical parameters of the ellipsoidal reflector. This type of shock wave is still used in lithotripters today, and the shock‐wave source of the HM3 is still considered to be the most effective of this type. Electrohydraulic shock waves have the disadvantage, in that the life span of the electrode tips is limited to several thousand shocks. Degradation of the electrode tips causes output variations and instabilities of the focus position, particularly near the end of the electrode’s lifetime. To compensate for the degradation of the electrode tips which causes variations and instabilities of the focal position and energy and limits the life span of the electrode to several thousand shocks, Technomed developed a modified electrohydraulic generator, the Diatron IV, an electroconductive shock‐wave generator. The electroconductive generator is a 100 nF generator connected to an electrode immersed in a highly conductive solution instead of degassed water. Due to a better conduction of electricity this highly conductive solution allows an extremely accurate spark position. This combined with reduced interelectrode distance leads to a reproducible and consistent focal spot. This spark gap technology also allows the generation of a focal zone that varies in size with the power output. An automatic pressure regulator permanently adapts the voltage input to consistently deliver the requested pressure to compensate the variations due to electrode wear and also controls the total energy delivered to the patient. The electromagnetic shock‐wave emitter (EMSE; Dornier, Siemens) features a flat coil that generates a strong magnetic field when a high current pulse flows through it. This field causes an isolated metallic membrane to be repelled into the surrounding water. This causes the water in the vicinity of the membrane to be compressed and a plane acoustic pulse is released, which is focused into the therapeutic focal area by a lens. The pressure across the membrane surface is essentially constant. During propagation to the focal area the pressure pulse undergoes nonlinear effects, leading to the generation of a shock wave. Specifically, the pulse’s rising slope becomes steeper and the pulse duration becomes shorter. Since the electromagnetic shock‐wave generation principle does not rely on the dielectric breakdown of a liquid, all the associated threshold effects and arc‐forming instabilities are avoided. An EMSE can therefore produce effective pressure pulses over a very wide intensity range with a high degree of reproducibility. This wide dynamic range allows for different shock‐wave treatment strategies to be used. The instrument’s life time is in the order of 1 million pulses. An EMSE does not show progressive degradation in its output power but it needs to be exchanged on a regular basis when the insulating materials and the metals have reached their endurance limits. The plane acoustical pulse generated by the EMSE is focused by an acoustic lens. The choice of the diameter of the EMSE and the focal length of the lens define the aperture angle of the system. The wide dynamic range that is available for EMSE systems, together with the wide choice of aperture angles, provides a unique toolbox for the designer, serving the different needs of shock‐wave field shaping in lithrotripsy, in orthopaedic shock‐wave application, and in other emerging applications. An EMSE is easily incorporated into a multifunctional device. Newer EMSE designs provide treatment results comparable to or even better than the results with the unmodified Dornier HM3. There is ongoing research to improve the performance of electromagnetic lithotripters through modification of the lens geometry [12]. The electromagnetic cylinder source was also developed to overcome some drawbacks of the powerful but unstable spark gap technology with a short life span of the eroding electrodes. The cylinder generator is based on a hollow electromagnetic cylinder composed of a cylindrical coil covered by an insulating membrane, which again is covered by an electrically conducting membrane of high strength. An intense pulse current with short rise time generates repelling pulse forces due to electromagnetic induction. When immersed in water the radially expanding membrane generates, primarily, an acoustic cylinder wave which is focused by a special rotational parabolic reflector. The principle works on the same basis as a loudspeaker, but with very intense and short acoustic pulses. The cylindrical coil/membrane configuration is very stable and delivers more than 1 million pulses without degradation or significant fluctuations of acoustic energy. The parabolic reflector can be designed with large apertures and, accordingly, large aperture angles without technical limitations. Due to the large aperture angle, the acoustic energy can be concentrated to laterally narrow and axially short focal zones with high peak pressure and high energy flux density values. Thus, the fragmentational shock‐wave energy is restricted to a relatively small area, reducing tissue lesions in front or behind the target stone. Also, anesthesia requirements and shock‐wave load affecting the coupling area at skin level are minimized. Contrary to the electrohydraulic spark gap technology, the energy level of each single shock‐wave pulse can be precisely adjusted between very low (painless) and powerful settings, exactly matching the pain tolerance of the patient. The generator configuration featuring a hollow cylinder provides sufficient space for favourable inline localizations either for fluoroscopic or ultrasonic targeting [13–15]. Piezoelectric shock‐wave generation was developed simultaneously by EDAP and Wolf [16–20]. The conversion of electrical to acoustic energy takes place in ceramic platelets that expand or contract in the surrounding water because of the piezoelectric effect when a pulse of several thousand volts is applied. Several dozen to several thousand platelets are usually mounted on a spherical segment transmitting the shock wave into the center of the sphere through direct focusing. However, compared to other generation principles the intensity of a typical piezoelectric generator is lower and a large converter surface is required to mount the platelets. The diameter of such a shock‐wave source is therefore large, providing sharp focusing of the acoustic pulse. The large diameter of the piezoelectric dish makes its incorporation in a multifunctional device more problematic, however. The piezoelectric shock‐wave source of the Wolf Piezolith 3000 features a double layer of piezo‐ceramic elements, known as Double Layer Piezo‐technology. Each type of shock‐wave source produces a shock wave, i.e. an acoustic pulse of which the energy is focused towards and concentrated in the therapeutic focus. In an electrohydraulic lithoptripter the focusing is achieved by a semi‐ellipsoid reflector, whereas in an electromagnetic lithotripter shock waves are focused using an acoustic lens (Dornier, Siemens) or a parabolic reflector (Storz). In a piezoelectric system there is no focusing as such. Each ceramic crystal is to be considered as a separate point source that produces its own miniature shock wave. The ceramic crystals are arranged on a dish that directs the point sources towards the therapeutic focus, where the accumulation of the energy of all these separate point sources produces sufficient energy for stone fragmentation: direct‐focusing lithotripsy (DFL). In a recently published experimental in vitro study bursts of high‐intensity ultrasound are used to disintegrate artificial and real urinary calculi. The conclusion of the in vitro study was that in vitro burst‐wave lithotripsy is feasible. The clinical use of this novel and interesting technique will of course depend on in vivo clinical studies proving both efficiency and the exclusion of adverse tissue effects [21, 22]. A shock wave is an acoustic pulse characterized by a very fast rise time, a very high overpressure, a very short pulse duration, and a phase of underpressure. The physics of shock waves being very complex [11], a basic understanding of some of the parameters in shock‐wave physics is useful (see also Chapter 58 in this volume). Only the more clinically relevant parameters will be discussed here. The maximum treatment depth is the distance between the shock‐wave head and the point of highest pressure in front of the shock‐wave head. This is the geometrical focus. The shock‐wave focus, however, is cigar shaped, with the axial dimension longer than the lateral. This means that there is still energy that may be sufficient to break a stone beyond the geometrical focus. This is referred to as the “blast path.” In their published specifications some manufacturers may “extend’ their treatment depth by also incorporate the blast path, thus adding half of the focal length to the geometrical focus and “stealing” some distance. There is an ongoing debate on the ideal focus size in a lithotripter [21, 22]. The ideal focus would be a spherical volume exactly matching the size of the targeted stone, thus limiting the administration of energy to the stone and avoiding hitting the surrounding tissue, which would lead to adverse tissue effects [21, 22]. This is technically not feasible, however. Today’s lithotripters have a cigar‐shaped focus with energy delivered in front, behind (blast path), and adjacent to the target. A small focus is highly confined and sharp. The higher pressure in a small focus leads to high efficiency in fragmenting hard stones. In order to obtain optimal fragmentation and to avoid adverse tissue effects, precise targeting is essential. In a large focus pressure is lower. Precise targeting is less critical but there is an increased analgesia need and a higher risk of adverse tissue effects. Sometimes there is a demand for larger focal zones in order to treat larger target volumes even if surrounding tissue may be affected more than technically necessary. To respond to this demand Storz introduced dual‐focus technology. The design of the electromagnetic cylinder source offers the possibility to select different focal zones, precise and extended, according to clinical and anatomical conditions. By modification of the electrical excitation of the coil, longer pulses may be genereated which, in turn, stretch the focal zone, both laterally and axially. The Wolf Piezolith 3000 features a triple focus: F1, small focus with high energy density (ED); F2, intermediate focus with medium to high ED; F3, larger focus with medium ED. Prospective trials would be useful to document the clinical benefits of selectable foci. It is generally assumed that a combination of four different mechanisms is involved in the interaction between shock waves and the targeted stone: the Hopkinson effect, cavitation, quasistatic squeezing, and dynamic fatigue [7]. It is also assumed that cavitation and shear forces (the Hopkinson effect) are the main culprits in the origin of adverse tissue effects. Although both shear forces and cavitation are important and necessary in the stone comminution process, avoidance of the negative effects of shear forces and cavitation is considered important in reducing adverse effects due to shock waves. The idea behind the dual‐pulse technique [23, 24] is to “fill” the negative part of the pressure pulse with a positive pressure pulse. In the Direx Duet dual shock‐wave sources are used. Fired synchronized or asynchronized they allow an increase in the total pressure and the shock‐wave rate. Sheir et al. [23] demonstrated improved stone comminution with a synchronous discharge of a twin pulse. In another study [24] the same group demonstrated higher performance and efficacy with this twin‐head, twin‐pulse technique. At the same time this technique produced far less parenchymal damage than with the use of a single treatment head. Theoretically this would reduce cavitation and improve disintegration. In ongoing efforts to engineer better lithotripters several studies have been and are being conducted to control cavitation and reduce its adverse tissue [25–28]. Eisenmenger and colleagues [29–31] advocate the use of a wide focus with lower pressure to enhance quasistatic squeezing, as this would presumably reduce tissue trauma and improve disintegration. This low‐pressure, wide‐focus technology is incorporated in the electrohydraulic Lithospace from Jena Med Tech GmbH (Jena, Germany) and the electrohydraulic LithoGold LG‐380 from MTS (Konstanz, Germany). In a study by Bhojani et al. [32] comparing the electromagnetic Modulith SLX to the LithoGold LG‐380 stone‐free rates proved modest with both devices using SWL alone. Outcomes with both systems also proved similar. In this study an advantage of the wide‐focus, low‐pressure principle could not be demonstrated. Further prospective clinical studies to corroborate (or disprove) this concept are eagerly awaited. Shock waves are acoustic waves that travel through a medium by alternating decompression and compression of the medium. Absorption, reflection, or refraction of the shock waves can occur at interfaces between media with different acoustic impedance. As water has comparable acoustical properties to human tissue, shock waves are generated and transmitted in water. In order to minimize energy loss of the shock wave at the interface between the patient and the shock‐wave source, coupling between shock‐wave source and patient is extremely important [31]. Total immersion of the patient in a water bath with degassed water where the shock wave is generated (Dornier HM3) is still considered the ideal coupling system. Second‐ and third‐generation lithotripters, however, feature “dry” coupling which consists of a water‐filled cushion that is inflated and pressed against the patient. The water cushion is made of elastic PVC or silicone, and is capable of adapting to the body shape of the patient. In order to guarantee good acoustic transmission, the water cushion is coupled to the patient through the application of ultrasound gel as an interface. In this process, both air bubbles in the coupling gel and folds in the water cushion could impair the transmission of acoustic waves. Even tiny air bubbles in this interface can lead to uncontrolled shock‐wave attenuation. In an in vitro experiment, Pishchalnikov et al. [33] found a reduction in fragmentation of 20–40% when air bubbles were included that covered an area equal to 2% of the cushion surface. Decoupling the cushion from the test basin and recoupling it led to a drastic increase in air inclusions. This has led to the recommendation that, if one decouples the patient during treatment, one must repeat the coupling procedure, including cleansing the coupling cushion and subsequently reapplying gel. Based on their own stone model, Jain and Shah [34] were able to identify a considerable influence of air inclusions on the erosion capacity of the shock wave. After experimenting with various techniques for gel application, as a method of choice Neucks et al. [35] recommend applying the gel as a bolus, specifically from a vessel with a large opening. A small vessel opening and manual distribution of the gel lead to increased air pockets and poor disintegration results. Bergsdorf et al. [36] also recommend the selection of a proper coupling gel disc or gel with low viscosity and the accurate removal of air bubbles to improve efficiency in SWL. In an experiment with an electromagnetic Dornier lithotripter equipped with an inline ultrasound scanner, Bohris [37] demonstrated that 43% more shock waves were needed to fragment model stones when only 8% of the coupling area, visualized with an inline ultrasound scanner, was covered with air bubbles. Li et al. [38] observed that the coupling interface is invisible during treatment and that the real problem is to avoid the entrapment of air bubbles during the coupling process. To monitor the coupling area in real time during stone treatments Bohris et al. [39] installed a video camera in the therapy head of a Dornier Lithotripter SII. In only 10 of 30 treatments could good coupling with an air ratio of less than 5% be obtained and in eight treatments the air ratio was higher than 20%. In a prospective clinical study using opical coupling control (see Figure 59.6 below) in a Dornier Gemini Tailly and Tailly‐Cusse [40] achieved comparable effectiveness quotients (EQ) with a reduction in shock‐wave numbers of 25.4% for renal and 25.5% for ureteral stones leading to a corresponding 25% reduction in treatment time. Energy levels could be reduced with 23.1% for renal and 22.5% for ureteral stones. In this study a video camera incorporated in the therapy head of a Dornier Gemini lithotripter allowed visual monitoring of the coupling area. Air bubbles that inevitably were trapped in this coupling area could easily be removed by gently swiping a hand between the patient and the water cushion, thus consistently achieving perfectly bubble‐free coupling. Adequate imaging is vital to the success of ESWL: Imaging modalities available on a lithotripter will to a great extent also define treatment strategies. Early lithotriptors were equipped either with ultrasound or fluoroscopy. In order to meet all the challenges in modern integrated stone management present day lithotriptors ideally should be equipped with both imaging modalities, to be used either independently and alternately or simultaneously. Inline use of both modalities is essential. Fluoroscopy allows targeting of radiopaque stones at all levels of the urinary tract. Direct targeting of radiolucent stones is impossible, however. Also smaller renal stones may prove more difficult to locate than with ultrasound. Further drawbacks are the absence of real‐time imaging and the exposure to radiation. With ultrasound it is generally impossible to locate stones in most parts of the ureter. On the other hand ultrasound allows direct visualization of radiolucent stones and offers easier targeting of smaller renal stones. A major advantage, however, is the real‐time imaging which provides better monitoring of the fragmentation process in the absence of exposure to radiation. Inline scanners are positioned along the shock‐wave propagation path and offer easier targeting of very proximal and very distal ureteral stones and easier dissociation between multiple stones. Image quality usually is poorer than with a lateral outline or free‐hand scanner, however, and rib shadows may hide stones from view. Outline scanners (free‐hand or mounted on a lateral isocentric arms) offer far better image quality and allow the selection of the most appropriate window to avoid rib shadows. Usually the appraisal of fragmentation is superior to an inline scanner. Proximal ureteral stones may be more difficult to find and targeting and positioning of prevesical stones is more cumbersome. To enhance targeting precision several manufacturers offer autopositioning and/or tracking systems: Dornier, EDAP TMS, Jena Med Tech, Storz, and Wolf. Machines where both ultrasound and X‐ray are integrated offer the most versatile imaging and targeting possibilities. Ideally these machines offer simultaneous online use of both ultrasound and X‐ray. Targeting versatility is further improved by the possibility to couple the therapy head to the patient both under and above the treatment table. Improved imaging and improved targeting will have a positive effect on the EQ. In the years following its introduction ESWL was the privilege of high‐volume stone centers. This was mainly due to the high capital, running, and maintenance costs of the first lithotripters. Only “dedicated” to extracorporeal lithotripsy these machines could only be operated in large centers with a high volume of stone patients. The construction of less expensive second‐ and third‐generation lithotripters with lower capital cost and lower running and maintenance costs made extracorporeal lithotripsy available in more and more centers, leading to a reduction of the number of patients treated per stone center. Stone therapy guidelines from international expert boards consider SWL in most types of stones as the first choice of treatment. However, in some indications endourologic procedures give equivalent or better results than SWL. Modern lithotripters are supposed to support urologists in any of these modalities. Consequently, in the past two decades lithotripters have undergone a transition from pure shock‐wave‐generating devices to multifunctional urologic workstations. One of the key components of such urologic workstations is the patient table. An increasing number of obese patients demands high‐load‐capacity tables with maximum accessibility in order to be able to approach the patient from all sides. For fluoroscopic imaging both in SWL and endourologic procedures the tabletop needs to be radiotransparent. Modern tabletops are therefore made of carbon fiber. Imaging modalities in a multifunctional workstation need to support the wide range of therapeutic options in urology. Large image intensifiers up to 40 cm ideally offer a large field of view of nearly the entire urinary tract. Full digitization of the X‐ray imaging chain provides excellent image quality at low radiation doses. In the newer devices flat‐panel detectors (FPDs) replace the traditional image intensifiers. Increasing awareness of radiation safety and dose reduction make ultrasound stone localization an ideal tool for stone targeting and accurate therapy monitoring. Last but not least, a high‐performance shock‐wave source, which ideally can be coupled to the patient in an over‐ and undertable position, completes the design of a multifunctional lithotripter. Multifunctional machines can have a modular design, where all components are independent and connected according to need, or an integrated design, where all components are integrated in the machine and ideally adapted to their function. A third design, the “hybrid,” offers integration of imaging and/or therapy head in a common console with an independent treatment table. In a lithotripter with a modular design (Figure 59.1) the different components, shock‐wave source, imaging components, and treatment table are separate modules to be connected to perform ESWL or endourological procedures. As the imaging components, both a fluoroscopic C‐arm and an ultrasound machine are usually available on site; investment cost is thus lower and limited to the shock‐wave module. The imaging components can also be used for other purposes both in urology and other departments. Modular systems have no need for a dedicated lithotripsy room and are easily transported from one center to another. Figure 59.1 Modular design: Jena Med Tech Lithospace. Reproduced with permission of Jena Med Tech GmbH. Due to the ad hoc combination of different modules the footprint is larger and the floor is cluttered with an array of machinery. In endourologic procedures this footprint is still enlarged by the addition of an electrosurgical unit, light sources, and monitors, etc. The uro‐table is less urologist‐friendly with limited accessibility. Overall handling is more complicated and less user‐friendly. In an integrated design the different components are fully integrated in one stand alone system (Figures 59.2–59.4). These systems have a small footprint compared to the modular and hybrid designs. They are usually high‐end devices ideal for centers with a sufficient volume of patients for SWL and endourology. Due to the optimal integration and synchronization of their high‐end components to a synergistic system these machines offer maximal versatility in targeting and positioning for SWL. Uro‐table function usually is excellent and offers great comfort in the performance of endourologic procedures. Ideally the patient table is entirely radiotransparent, accessible over 360°, and capable of bearing obese patients. The high investment cost and the fixed installation in a dedicated endourology/ESWL room may prohibit its acquisition in centers with limited patient loads. Figure 59.2 Integrated design: Dornier Gemini. Reproduced with permission of Dornier MedTech GmbH. Figure 59.3 Integrated design: Storz Modulith SLX‐F2. Reproduced with permission of Storz Medical AG. Figure 59.4 Integrated design: EDAP TMS Sonolith i‐Sys. Reproduced with permission of EDAP‐TMS France. In these machines imaging (fluoroscopy and/or ultrasound) and shock‐wave source are mounted on a combined console (Figure 59.5). The patient treatment table is a stand‐alone component to be added to the system for SWL or endourological procedures. Both investment cost and footprint are medium, i.e. between that of a machine with integrated design and a device with modular design. These systems are easily transportable and do not need a dedicated room. Some of the components (imaging, patient table) are suited for multifunctional and multidisciplinary usage. Uro‐table function usually is adequate and overall handling is in between the modular and the integrated design. Figure 59.5 Hybrid design: Wolf Piezolith 3000 plus. Reproduced with permission of Richard Wolf GmbH. To measure the performance of a lithotripter, formulae have been devised that take into account stone‐free rate, retreatment rate, and auxiliary procedure rate. The original effectiveness quotient (EQA) was defined by Denstedt, Clayman and Preminger [7, 41]:
Lithotripsy Systems
Historic perspective
Components of a lithotripter
Shock‐wave sources
Electrohydraulic shock‐wave sources
Electrohydraulic shock‐wave generation
Electroconductive shock‐wave generation
Electromagnetic shock‐wave sources
“Flat coil” electromagnetic shock‐wave emitter
Storz electromagnetic shock‐wave source: cylinder source with parabolic reflector
Piezoelectric shock‐wave sources
Burst‐wave lithotripsy
Introduction to shock‐wave physics
Maximum treatment depth or penetration depth
Clinical relevance of focus size
Shock‐wave interaction mechanisms
Coupling
Imaging systems
Lithotripter design
Dedicated or multifunctional machine?
Integrated or modular?
Modular design
Integrated design
Hybrid design
Performance of lithotripters
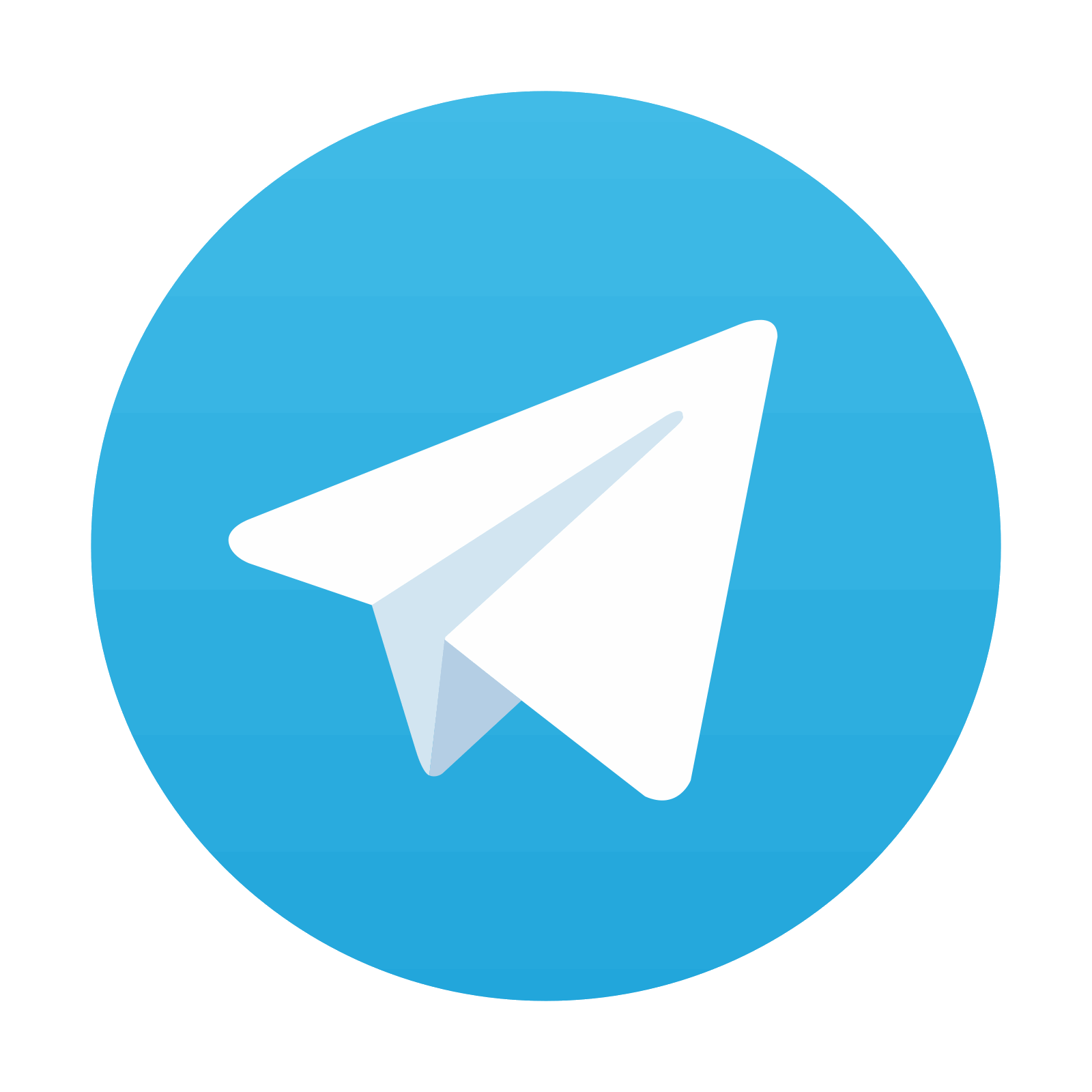
Stay updated, free articles. Join our Telegram channel
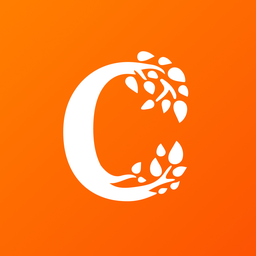
Full access? Get Clinical Tree
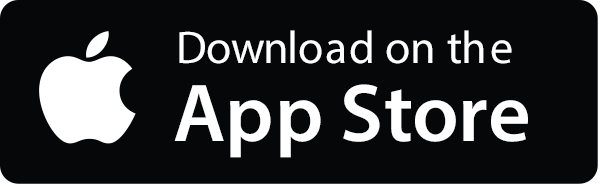
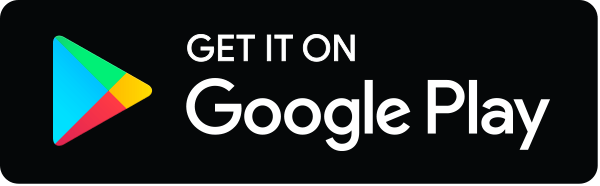