144 Christopher Netsch,1 Bilal Chughtai,2 Alexis E. Te,3 Ahmed M. Elshal,4 Mostafa M. Elhilali,5 & Andreas J. Gross1 1 Department of Urology, Asklepios Klinik Barmbek, Hamburg, Germany 2 Department of Urology, Weill Cornell Medical College, New York Presbyterian Hospital, New York, NY, USA 3 Weill Medical College of Cornell University, New York, NY, USA 4 Urology and Nephrology Center, Mansoura University, Egypt 5 Division of Urology, McGill University, Montreal, QC, Canada In his publication on quantum theory in 1917, Albert Einstein postulated the existence of extremely focused light beams [1], but it was another 43 years before the first commercially available laser became available, as published by Maiman [2]. The acronym LASER was created by Gould in 1957; it stands for laser amplification by stimulated emission of radiation [3]. In simple terms, a laser is a parallel light of a specific wavelength and direction [4]. Immediately after its first description, manifold lasers were developed and were widely used in many fields of medicine. Most of them did not stand the test of time, however, and the unsuccessful clinical application of laser devices led to a general decline in their use during the 1980s. Ten years later, an improved understanding of laser principles provided better knowledge that led to the selection of appropriate lasers for specific medical applications [3–7]. With the development of lasers with many different wavelengths and qualities, various laser systems that could be used to treat urological conditions were developed. This chapter reflects the cumulative understanding of several different urological surgeons on the most widely used laser systems at this time. An important facet of the clinical application of lasers is the optimization of the absorption process of light energy in tissue, with the knowledge that laser radiation is a directed light of a narrow bandwidth with specific effects. Each laser is synonymous with a single color or electromagnetic wavelength, and each laser can be derived from many wavelengths of the invisible and visible electromagnetic spectrum [8]. Laser light is created by a quantum mechanical principle of “stimulated emission” of radiation in an excited state (active media: gas, crystal, glass, dye). Excitation of the laser medium can be achieved in various ways (e.g., excitation by photons from a flashlamp). Some of the excitation photons are absorbed by the active medium, leading to an excited state [4]. According to the theory of spontaneous emission, these excited states return to the ground state, statistically releasing a photon at a characteristic wavelength. If these spontaneously emitted photons hit another excited state of the same excitation energy, it stimulates the excited ion to return to the ground state by emitting a further photon with the same wavelength and the same direction of propagation and the same phase. This is called stimulated emission of radiation (Figure 144.1) [4–7]. The emitted wavelength is characteristic for the optically active components of the laser medium [4]. Figure 144.1 Spontaneous and stimulated emissions of radiation. Source: Bach T, Muschter R, Sroka R et al. Laser treatment of benign prostatic obstruction: basics and physical differences. Eur Urol 2012;61:317–325. Reproduced with permission of Elsevier. To achieve controlled excitation of the laser medium, it is embedded into an optical resonator, a set of mirrors within the optical axis of the laser medium that redirect emerging photons into the laser medium for further amplification. One of the resonator mirrors has a reflectivity at the laser wavelength of nearly 100% (high reflector) and the other one is partially transparent to allow laser radiation to exit from the resonator (output coupler). Laser radiation is emitted from the resonator in a continuous wave or pulsed manner depending on the construction of the laser device (e.g. excitation source, characteristics of the laser medium) or the presence of additional optical elements, such as a Q‐switch [4]. Q (or Quality)‐switching is a technique to generate short, high‐peak, powerful laser pulses by modulating the cavity Q‐factor (cavity loss) of the laser resonator. This comprises an optoelectronic shutter that is embedded within the laser resonator, which is periodically opened and closed. When it is closed (low cavity Q‐factor), stimulation of emission is suppressed, but the population inversion reaches a large value due to the ongoing excitation process. When the shutter opens (high cavity Q‐factor), spontaneously emitted photons start oscillating within the resonator, leading to an avalanche of stimulated emission. The larger the population inversion, the higher the Q‐switch pulse peak power. The Q‐switch pulse terminates with depletion of the population inversion [4]. Second harmonic generation (SHG) is a second‐order nonlinear process used to convert a given laser (used in the potassium titanyl phosphate (KTP) laser) to half of its fundamental wavelength (second harmonic). It comprises a nonlinear crystal that needs to be carefully matched with the fundamental laser wavelength. Sufficient power density of the fundamental laser and exact alignment of the nonlinear crystal with the direction of propagation is required to generate second harmonic photons, which are double in frequency and/or half in wavelength [4]. In diode lasers, a semiconductor laser diode is used to generate laser radiation. The semiconductor material defines the wavelength. Electrical current flow is used to stimulate the semiconductor, which emits laser radiation after exceeding the threshold. Diode laser systems achieve a high electrical‐to‐optical energy conversion efficiency [4]. In contrast to diode lasers, other commonly used laser systems (holmium, thulium, GreenLight) utilize a crystal as the laser medium. In these laser systems the active laser ion is doped into the yttrium–aluminum–garnet (YAG) crystal that serves as a carrier for rare earth material defining laser characteristics. The widespread use of the YAG crystal is rooted in its physical properties. Crystals with garnet structure offer three crystallographic sites of different size and geometry, {C3}(A2)[Al3]O12, that are suitable for doping with optically active transition metal and rare earth metal ions. YAG is isotropic and has a broad range of spectral transparency. The YAG crystal also offers excellent mechanical and thermal properties. It is easy to grow following the Czochralski technique, which involves growing single crystals from their melt. During the growth process, the molten material is kept in a heated crucible. A seed crystal is lowered from above until it reaches the melt surface. The crystal boule is grown by pulling the seed crystal slowly upwards under continuous rotation [4–7]. For these reasons the YAG crystal is the material of choice for a variety of solid‐state laser materials. By replacing a percentage of the yttrium (Y3+) ions at the crystallographic C‐position (doping) with three valent rare earth ions such as neodymium (Nd3+), holmium (Ho3+), thulium (Tm3+), or erbium (Er3+), optically active solid‐state laser materials may be created. Under excitation, these crystals have the ability to amplify their own fluorescence light by stimulated emission of radiation [4–7]. Optical fibers deliver laser radiation to the target safely and efficiently, although several issues have to be resolved. Laser radiation has to be coupled from the generator to the fiber and transported within the fiber to the desired point of application [4]. A laser fiber includes a circular fiber core and two or more levels of fiber cladding clasped around this core. Laser radiation is delivered through the circular fiber core, whereas the adjacent layer provides entrapment of laser radiation within the core and the remaining layers conserve fiber integrity [1, 6]. The optical core is made from silica. Standard silica material is used effectively for visible light, but the transmission of 2 µm radiation by flexible silica laser fibers, as in holmium and thulium lasers, requires purified low hydroxide (OH) silica to eliminate power loss during the delivery due to energy absorption in the OH− ion [6]. To suppress leakage of laser radiation, the core is surrounded by an optical cladding made from a transparent material of a lower refraction index. Fluorine‐doped silica cladding is considered the primary choice for high‐quality fiber applications. Less flexible fluoroacrylate cladding (TECS) is used for fibers to transmit shorter wavelength and low‐power applications [5]. To launch laser radiation into an optical fiber, a focusing lens with a receptacle for the fiber connector is necessary. Connecting the fiber to the laser generator is critical for energy transmission [5]. A slight deviation of the focal point of the fiber coupler lens leads to laser radiation absorption by the connector, which guides the laser fiber to the coupler lens. Thus it will result in heating of the connector. At sufficiently elevated power levels, the focused laser beam will melt and vaporize, resulting in destruction of energy transmission [4]. Independently of the fiber type, the laser beam is delivered through the optical core of the fiber [4–7]. In side‐firing fibers, the reflection of the laser beam is achieved by an oblique surface at the fiber tip where the radiation is reflected sideways either by total reflection of the beam on a core–air interface or an additional reflective coating. This deflects the laser beam toward the tissue at an oblique angle of about 70°, which is provided by the physics of total reflection at an internal glass–air surface. By sweeping the beam and changing the distance between fiber tip and tissue surface, coagulation as well as vaporization can be achieved. The pitfalls of side‐firing fibers are the limited lifespan due to exposure to heat that is generated by carbonization at the laser beam output [6], leading to fiber damage and consequently to loss of efficacy [5, 9]. However, continuous flushing of the beam exit with cooler irrigation fluid alleviated this effect as designed in the MoXy™ fiber. By increasing the optical core diameter and the introduction of liquid cooling as well as a metal capping of the fiber tip, the lifespan of the side‐firing fiber seems to be increased [10]. Although it is generally plausible to manufacture a MoXy‐type side‐firing fiber for all laser systems, this design is currently applied only with the GreenLight XPS™ system [4]. Laser radiation discharges from the tip into the tissue by rapid heating of the tissue in a very small tissue volume. Tissue effects such as vaporization and disruption can be achieved. Another approach called twister fiber is used for contact laser vaporization, as introduced by Biolitec for its laser system. The Biolitec laser uses a wavelength at which water is included as a chromophore. As a result, in front of the fiber tip a steam bubble is created, within which vaporization is achieved; vaporization is also created by the secondary heated button‐shaped fiber tip [4]. A near‐contact technique is required. Laser radiation is emitted from the resonator in a continuous wave (cw) or pulsed mode depending on: Holmium lasers are normally excited by flashlamps, which restricts the repetition rate due to heat accumulation in the laser crystal. In contrast, Tm:YAG lasers may operate in a cw‐mode if excited by a laser diode. Pulsed holmium lasers create large pulsating steam bubbles in the irrigant surrounding the tip of the laser fiber, whereas the bubbles are much smaller for cw‐mode lasers (Figure 144.2a,b). For the holmium laser, the lifetime of the steam bubble corresponds to the duration of the laser pulse (~500 µs) which is too short to be visible in video transmission during endoscopic procedures [8]. Figure 144.2 (a) A large steam bubble forms at the end of a 550 µm laser fiber when using a Ho:YAG laser. (b) A small, relatively constant steam bubble forms at the end of a 550 µm laser fiber when using a Tm:YAG laser.
Laser in Benign Prostatic Hyperplasia Treatment: Basic Principles
Introduction to lasers
Generation of a laser beam
Delivery of laser radiation
Laser fiber design
Types of fiber endings
Side‐firing fibers
End‐ or front‐firing fibers
Twister fibers
Pulsed and continuous wave lasers
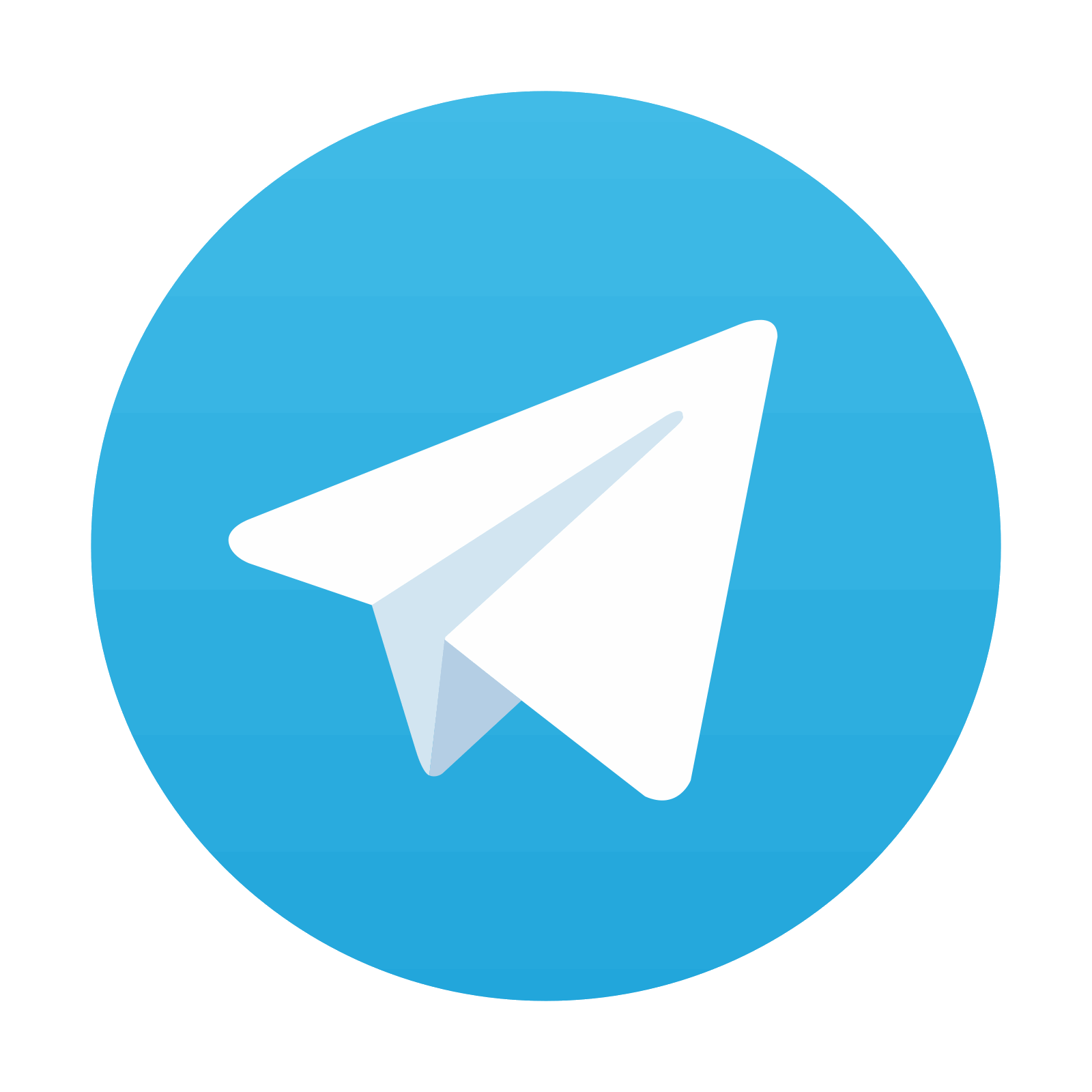
Stay updated, free articles. Join our Telegram channel
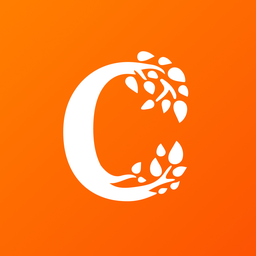
Full access? Get Clinical Tree
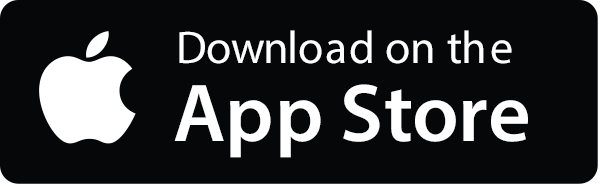
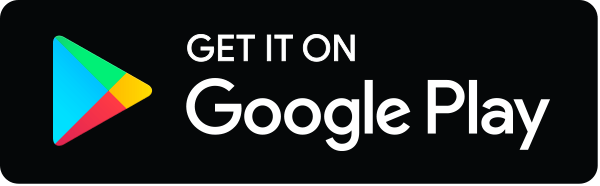