6
Kidney and Ureter Physiology
Motaz ElMahdy Hassan1 and Mohamed Ismail2
1 NHS Grampian, Aberdeen Royal Infirmary, Aberdeen, UK
2 Portsmouth Hospitals, NHS Trust, Portsmouth, UK
Abstract
The kidneys play an important role in regulating the internal environment of the human body. They eliminate waste products, maintain fluid and electrolyte balance, and have a hormonal function. Kidneys ensure that the internal environment is maintained for normal function of the body. They also secrete various substances that regulate bone metabolism, vascular tone, and haemopoiesis. Understanding renal physiology is essential to understanding disease process and providing clinical care to patients.
Keywords: glomerular filtration rate; acid–base balance; acidosis; renal tubule; erythropoietin; vitamin D
6.1 Glomerular Filtration Rate
Kidneys are supplied by renal arteries that branches directly from the abdominal aorta. Renal arteries divide progressively, leading to a glomerular capillary plexus. The glomerulus is the functional unit of the kidney. In a normal physiological condition, 20% of the cardiac output (625 ml min−1 per kidney) flows through the 2.5–3 million glomerular capillary plexus, losing about 170 l of plasma water per day, completely recycling it every 30 minutes and the whole body water four times a day [1].
The fenestrations of the capillary gives the plasma direct access to the basement membrane whose effective mesh has been measured with molecules of known molecular weight, (40 000 mol wt), that is, the glomerular filtrate is plasma minus its proteins [1].
Filtration depends not only on the size of the molecule but also on its electrical charge. The proteins of the glomerular basement membrane carry a negative charge which repels negatively charged protein molecules (e.g. albumen). The blood pressure within the glomerular arteriole is about 60 mm Hg. The plasma oncotic pressure is about 25 mm Hg, so that there is a filtration pressure of about 35 mm Hg (Figure 6.1).

Figure 6.1 Filtration in the glomerular arteriole.
Glomerular filtration rate (GFR) is the rate of filtered fluid through the kidneys per unit time. It is controlled by three factors: (i) the difference in hydrostatic pressure and oncotic pressure between the capillary membrane and Bowman capsule, (ii) the renal plasma flow, and (iii) the glomerular permeability, which is the least important factor [2].
Vascular tone of the afferent arterioles changes in response to the change in the mean arterial pressure, a mechanism called ‘autoregulation’. Therefore, GFR is maintained at a constant level despite the variation in the systemic blood pressure. Autoregulation becomes less effective in controlling GFR when the mean arterial pressure drop below 70 mm Hg. A feedback mechanism from the macula densa in response to the sodium and chloride excretion has also been suggested [3].
GFR can be measured by various methods [4]. The gold standard is inulin clearance. Inulin is a fructose polysaccharide that is filtered by the glomerulus but is neither absorbed nor secreted in the renal tubule, which are the key points to adequately measuring the GFR. Inulin is infused intravenously until a steady state is reached. Its concentration is measured in blood and urine collected over a timed period.

where U is the urine concentration of inulin, V is the volume of urine per minute, and P is the plasma concentration of inulin.
Inulin is difficult to administer and difficult to measure; therefore, it is not routinely used in clinical practice. The most widely used method to estimate GFR is creatinine clearance. Creatinine clearance rate is the volume of blood plasma that is cleared of creatinine per unit time. It is easy to perform, relatively cheap, and readily available. The endogenous creatinine is produced at a constant rate with limited daily variation. This method assumes that creatinine is neither excreted nor reabsorbed in the renal tubule. Nevertheless, some of the creatinine is excreted in the proximal tubule, which overestimates the true GFR [5]. When GFR is very low, measured creatinine clearance can exceed true inulin clearance by 50–100% [6].
In the clinical practice, there is a major practical drawback to the measurement of creatinine clearance in that it demands an exact timed collection period. Usually, the patient passes urine before going to sleep and notes the time. In the morning the bladder is emptied and the time is noted again; the overnight urine specimen plus a blood sample taken early in the morning is sent to the laboratory [7, 8]. This system only works when the patient is reasonably well and cooperative, which is not always the case.
Isotope compounds can also be used to measure GFR such as 51Cr‐ethylenediaminetetra‐acetic acid (EDTA), diethylenetriaminepentaacetic acid (DTPA) labelled with 140La or 99technetium. These substances are much more efficient and accurate, although more expensive [9].
Measuring plasma level of urea and creatinine is the most widely used method to estimate GFR. Creatinine levels vary between individuals and an estimated GFR (eGFR) can be calculated based on patient age, sex, and body mass using the following more common formulas [2].
- Crockcroft‐Gault formula: eGFR = (140‐Age) × Mass (Kg) × [1.04 if female and 1.23 if male]/Serum Creatinine (μmol l−1) or eGFR = (140‐Age) × Mass (Kg) × [0.85 if female]/72 × Serum Creatinine (mg dl−1)
- Modification of diet in renal disease (MDRD) formula: eGFR = 32 788 × Serum Creatinine−1.154 × Age−0.203 × [1.21 if Black] × [0.742 if female] or eGFR = 186 × Serum Creatinine−1.154 × Age−0.203 × [1.21 if Black] × [0.742 if female]
(Creatinine levels in μmol l−1 can be converted to mg dl−1 by dividing them by 88.4).
6.1.1 Disorders of Glomerular Filtration
Glomerular filtration may be impaired in underperfusion from dehydration, low blood pressure, septicaemia, cardiac failure, hepatorenal failure syndrome, and nephrotoxic drugs.
6.2 Renal Tubules
Renal tubules have two main functions: secretion, which is the removal of substances from the blood into the lumen, and reabsorption, which removes substances from the lumen to the blood. Renal tubules extend from the renal cortex to the inner medulla, and it is subdivided into different sections each has specialised function.
6.2.1 Proximal Convoluted Tubules
Proximal convoluted tubules (PCTs) extend from the Bowman capsule to the loop of Henle. PCT is responsible for reabsorption of 60% of the glomerular filtrate including 80% of water. Sodium, potassium, calcium, and bicarbonate ions are mainly reabsorbed in the PCT. All of the filtered amino acid and glucose are also recovered in here. PCT is responsible for the secretion of drugs and toxins that are too large to be filtered in the glomerulus. Most common diuretics act on the proximal tubule to reduce sodium and water reabsorption.
Sodium reabsorption occurs by means of passive and active process. Passive reabsorption accounts for 15% of the total sodium reabsorption and is driven by the electrochemical gradient created by chloride anion [10]. The active mechanism of sodium reabsorption is driven by a sodium/potassium ATPase pump. It is an energy‐dependent mechanism which moves three sodium ion into the peritubular capillaries and two potassium ion in the opposite direction. Water will passively follow sodium ions. Glucose and amino acid will be cotransported with sodium ion.
The majority of filtered bicarbonate is reabsorbed in the PCT. Bicarbonate is coupled with the hydrogen ion to form water and carbon dioxide. Both will then diffuse passively and combine inside the cell to form a hydrogen ion and bicarbonate in a reaction facilitated by carbonic anhydrase enzyme. Most of phosphate and calcium ions are reabsorbed in the PCT.
6.2.2 Loop of Henle
The main function of loop of Henle is creating hyperosmolar medullary interstitium to facilitate urine concentration process in the collecting ducts. The loops of Henle can be subdivided into three parts: thin descending limb, thin ascending limb, and thick ascending limb (Figure 6.2). Each segment has a specified physiological function, and a specialised peritubular capillary plexus called ‘vasa recta’ surround the loops. The thin descending limb is highly permeable to water and relatively impermeable to salt. The thin ascending limb has no active transport of salt, and it is impermeable to water. The thick ascending limb is entirely impermeable to water. The reabsorption of solute in this segment occurs though an active process. [11].

Figure 6.2 The countercurrent hypothesis for the formation of hyperosmotic urine.
6.2.2.1 The Countercurrent Theory
Filtrate in the loop of Henle and blood flow in the peritubular capillaries are running in the opposite direction creating a countercurrent. In the thick ascending segments, sodium is removed from the filtrate into the cell through Na/K/2Cl transporter that is located in the apical membrane of the columnar epithelial cell. Sodium is then removed from the cell in exchange for potassium through an active process involving the Na/K ATPase pump, reducing the sodium concentration and osmolality of the filtrate and increasing them in the interstitial fluid (Figure 5.2) [12]. The filtrate, which flows down the descending limb, starts off being iso‐osmotic with systemic plasma and glomerular filtrate. As it descends through the hyperosmotic zone, water diffuses out and the filtrate becomes more concentrated. As it rises up through the thick limb of the loop of Henle, it brings a high concentration of sodium chloride to the active sodium/potassium exchange pump, and sodium is pumped out into the interstitial tissue, raising its osmolality still further. The ability of the sodium pump in the thick segment of the ascending limb of the loop of Henle to establish a modest concentration difference across the wall of the ascending limb is augmented by countercurrent flow to achieve a large difference in osmolality [13, 14].
Several control mechanisms are involved in this process of water conservation: the pituitary antidiuretic hormones, arginine vasopressin being the most important of these. Receptors for arginine vasopressin are found along the entire length of the renal tubule [13].
The atrial natriuretic peptide is a polypeptide secreted by the human atrium, which causes diuresis and loss of sodium. It is of interest to urologists because it is secreted whenever obstruction of urine is relieved. It may play a part in the diuresis which occurs when the bladder is catheterized for chronic retention. Both proximal and distal tubules take part in this postobstructive diuresis [15].
Diuretics, which prevent reabsorption of sodium in the proximal tubule, mean that more sodium reaches the distal tubule to be exchanged for potassium; hence, hypokalaemia can be seen after prolonged administration of diuretics.
6.2.3 Distal Convoluted Tubule
The distal convoluted tubule (DCT) extends from the loop of Henle to the collecting duct. It is essential for the reabsorption of sodium and calcium ions. It also form the macula densa, part of the juxtaglomerular apparatus that senses the change in sodium ion concentration. Ten percent of the filtered sodium is reabsorbed in the DCT via an active basolateral sodium potassium ATPase pump. Thiazide diuretics work on this part to inhibit sodium and chloride absorption and cause dieresis.
Unlike the other parts of renal tubules, calcium reabsorption occurs through calcium channels in the apical membrane and not through the paracellular route. Calcium ion reabsorption in this part is controlled by parathyroid hormone [16].
6.2.4 Collecting Ducts
Collecting ducts (CDs) are the final part of renal tubule and are divided into cortical and medullary ducts. CDs are lined by two types of cells. Principal cells reabsorb sodium and secrete potassium into the lumen. Intercalated cells are responsible for hydrogen and bicarbonate secretion and play a role in acid–base balance. Antidiuretic hormone is secreted from the hypothalamus to the posterior pituitary gland. It is released in response to increase in plasma osmolarity. It increases the permeability of the medullary part of the CD to water and increases urine concentration.
6.3 Hormonal Function of the Kidney
6.3.1 Renin
Renin is secreted by the juxtaglomerular cells in the zona glomerulosa, situated on the afferent arteriole of the glomerulus, in response to a fall in blood pressure [17]. Renin is an enzyme which splits angiotensinogen (a globulin) into angiotensin I (decapeptide) which in turn is converted to angiotensin II (a potent vasoconstrictor). Angiotensin II causes an increased cardiac output and constriction of the peripheral arterioles. Angiotensin II is important for maintaining GFR when the renal blood flow decreases. It also stimulates the secretion of aldosterone to conserve sodium and increase the total extracellular fluid volume.
6.3.2 Erythropoietin
Erythropoietin, a potent haematopoietic growth factor, is produced primarily by the kidneys. It stimulates division of erythroid colony forming units in the bone marrow to form red blood cells [18]. In renal failure, lack of erythropoietin results in anaemia, but fortunately synthetic erythropoietin, made by recombinant DNA technology, is available for patients on dialysis [19].
6.3.3 Vitamin D
The kidneys play a vital role in vitamin D metabolism. Vitamin D is synthesised in the dermis from cholecalciferol (vitamin D3). Vitamin D3 is inactive and requires two hydroxylation to become active. The first hydroxyl group is added in the liver to form 25‐hydroxycholecalciferol (calcidiol). The second hydroxyl group is added in the renal tubular cells to form 1,25 dihydroxycholecalciferol (calcitriol), the active form of vitamin D. The kidneys also control bone mineralisation through the reabsorption of calcium ion. Parathyroid hormone significantly increases calcium reabsorption in the distal tubule. It also reduces phosphate reabsorption in the PCT [2].
6.4 Special Disorders of Renal Tubules
Cystinuria is an autosomal recessive disorder. Mutation affects SLC3A1 and SLC7A9 genes on chromosome 2, leading to deficiency in transporter protein of four amino acids arginine, ornithine, lysine, and cystine [20]. The first three are highly soluble, whereas failure of cystine reabsorption in the proximal tubule results in recurrent stone formation.
6.4.1 Hartnup Disease
Hartnup disease is an autosomal recessive disorder in which the deficiency involves the transport of tryptophan across the renal tubule and bowel wall. The loss of tryptophan in the urine is not important, but reduced uptake from the bowel results in deficiency of nicotinamide, resulting in cerebellar ataxia and pellagra [21].
6.4.2 Fanconi Syndrome
In Fanconi syndrome, the proximal tubule is converted into a short, thin functionless tube. As a result, there is failure of reabsorption of glucose, several amino acids, and phosphate. There is usually proteinuria and acidosis [21].
6.4.3 Renal Glycosuria
In renal glycosuria, there may be incomplete reabsorption of glucose from the filtrate, resulting. Renal glycosuria is harmless, but it must be distinguished from diabetes [21].
6.4.4 Renal Tubular Acidosis
Renal tubular acidosis (RTA) is a disease that occurs when the kidney fails to secrete hydrogen ions, resulting in acidic blood and alkaline urine. There are four types of renal tubular acidosis. Type 1 (distal RTA) is characterised by the inability to secrete hydrogen ions, leading to an alkaline urine which predisposes to calcium phosphate stone formation, metabolic acidosis, hypocitraturia, and hypokalaemia. Type 2 (proximal RTA) is characterised by failure of bicarbonate reabsorption; however, it is also associated with increased urinary citrate excretion but does not form stones. Type 3 (variant RTA) is a combine proximal and distal types. Type 4 (hyperkalaemic RTA) is seen in patients with hypoaldosteronism or pseudo‐hypoaldosteronism (i.e. failure of kidney to respond to aldosterone) [22].
6.4.5 Nephrogenic Diabetes Insipidus
Nephrogenic diabetes insipidus results from the failure of the kidney to respond to antidiuretic hormone. It could be hereditary or acquired. The hereditary form is an x‐linked recessive disorder that affects males. The condition is characterised by excessive dieresis and subsequent dehydration [23].
6.5 Acid–Base Metabolism
The kidneys play an important role in acid–base metabolism (Figure 6.3). The majority of acid production in the body comes from carbohydrate, fat, and protein metabolism. Metabolic by‐product carbon dioxide is converted to hydrogen ion and bicarbonate. The majority of acid production is eliminated by the lung. However, fixed acid which cannot be eliminated by the lungs has to be secreted by the kidneys [2]. In the renal tubule, bicarbonate is formed from carbon dioxide and water in a reaction catalysed by carbonic anhydrase [24]. The majority of bicarbonate is reabsorbed in the PCT and the remainder is reabsorbed in the DCT. A hydrogen ion is secreted in the renal tubule in exchange with a sodium ion through Na+‐H+ pump. It is also secreted through H + ‐ATPases proton pump [2].

Figure 6.3 Diagram showing the mechanisms involved in regulating acid–base balance in the renal tubule.
In the distal tubule, glutaminase forms ammonia from glutamine, which combines with H+ to form ammonium.
The buffering systems are as follows:
Bicarbonate: H+ + HCO3 − ↔ H2CO3 ↔ H2O + CO2
Phosphate buffer: H+ + PO4 −3 ↔ H+ + HPO4 −2 ↔ H+ + H2PO4 −1 ↔ H3PO4
Ammonia buffer: NH3 + H2O ↔ NH4 + + OH−
6.6 Obstructive Uropathy
Obstructive uropathy is the most common correctable cause of renal failure caused by impaired flow of urine [25]. Characterised by three phases. In phase 1 (within 1.5 hours of unilateral post‐obstruction), the pressure rises inside the renal pelvis until the filtration pressure is exceeded and filtration ceases. The rise in pressure results in stretch of the tubular cells, which trigger a transient release of prostacyclin, prostaglandin E, and nitrous oxide. The net effect is a transient increase in the renal blood flow to maintain GFR by dilating afferent arterioles. In phase 2 (1.5–5 hours), efferent arteriole vasoconstriction mediated by angiotensin II and thromboxane A2 drops the renal blood flow, while the pressure continues to rise. In phase 3 (after 5 hours), angiotensin II and thromboxane A2 cause the afferent arterioles to vasoconstrict, which further reduces the renal arterial blood flow and also the pressure reduces. In bilateral obstruction, the third phase does not exist, which leads to continual increase in intrarenal pressure.
Within 24 hours, renal blood flow falls to only 40% of the preobstruction level [26, 27]. Hypoxia of the renal tissue promotes the synthesis and release of inflammatory mediators, which recruit macrophages and promote interstitial fibrosis and scarring.
Splitting of the renal tissue can occur because of increasing pressure, mainly at the edges of the calyces, which allows urine to enter the renal lymphatics and veins. This is demonstrated as stranding on radiological images.
There are two practical implications of this phenomenon. First, microorganisms in the upper tract can gain immediate access to the bloodstream (i.e. obstruction complicated by infection is easily followed by septicaemia). The second is that when urine finds its way into the lymphatics or veins of the kidney, the pressure inside the lumen of the renal pelvis will fall, and filtration will start again. Thanks to this safety‐valve mechanism, acute obstruction in the human kidney does not mean that the kidney ceases to function. An apparently nonfunctioning kidney may recover completely after the obstruction has been relieved.
Following the relief of obstruction, renal blood flow increases to 60% of the normal. GFR remains at 50% of its normal levels. Postobstructive diuresis is more common following bilateral renal obstruction. Postobstruction diuresis can occur, loosely defined as >200 ml of urine an hour. This is mainly a physiological process (90% of cases) because of the disruption of the medullary osmotic gradient, enhanced medullary blood flow, fluid overload, and diuretic effect of excess urea, which is adaptive and aimed at clearing out the accumulated fluids and metabolic wastes. Although 10% can be pathological as a result of secretion and accumulation of arterial natriuretic peptides (due to over stretching of the atria) in unilateral obstruction, excess ANP is excreted by the other kidney; furthermore, loss of antidiuretic hormone sensitive of the collecting ducts can also lead to large fluid losses. The diuresis can be clinically driven by administrating unnecessary large volumes of intravenous fluids during the recovery phase [15].
6.7 Ureteric Physiology
The ureter is not merely a conduit for urine but rather an organ that represents a high degree of integration with the kidney and the bladder by being responsive to both physiological and pathological changes [28]. The propagation of urine starts from the kidney with the pelvicalyceal system and the renal pelvis having greater frequency of contractions to push the urine into the upper ureter [29]. As the urine moves into the upper ureter to be propelled further, the contractile activity at the pelviureteric junction (PUJ) protects the kidney from reflux of urine from the upper ureter [29]. As urine flow increases, the block at the PUJ loses this effect, and a rhythmic contraction pattern takes place between the pacemaker activity in the upper parts of the ureter and the rest of ureter, hence producing an equal and continuous wave of ureteric contractions [30, 31]. The peristaltic wave originates in the most proximal portion of the ureter, and urine is propelled distally forming a bolus. For the bolus of urine to be propelled efficiently, the peristaltic wave must completely coapt the ureteral walls. The resting ureteral pressure is approximately 0–5 cm H2O and ureteral contractions that occur in response to urine flow, which is about two to six times per minute, induces further increase in pressure that can go up to 20–80 cm H2O [32].
The vesicoureteric junction (VUJ) allows the urine to enter the bladder as a one‐way transport of urine. The peristaltic wave forces the urine through the VUJ, and the energy of the contractile wave dissipates. In normal flow conditions, the ureter can transport a specific amount of urine per unit time. As the rate of flow increases, the ureter does not form a bolus and coapt but rather forms a continuous column of fluid, and the ureter serves as a simple pipe for transport (Figure 6.4).

Figure 6.4 In diuresis, the ureter dilates (a), the peristaltic contractions become less occlusive (b) until eventually the ureter acts as an open pipe (c).
Different conditions can affect the adequacy of urine transport. Inadequate transport can result from increased or reduced amount of fluid entering the ureter per unit time. If the rate of flow is quite high, a normal nonobstructed ureter may dilate and impede adequate urine transport. Also a minor degree of obstruction to outflow may not cause significant dilatation at low flow rates.
Urine transport across the VUJ is dependent on the relationship between the ureteral intraluminal pressure and intravesical pressure. During normal flow rates, the ureteral contractile pressure exceeds the intravesical pressure with the urine passing into the bladder. In a case when the ureter is dilated and inadequately contracting or at very high flow rates, the ureter does not coapt to form a bolus and the pressure in the column of urine within the ureter must become higher than the intravesical pressure for urine to pass into the bladder. The normal bladder maintains a relatively low intravesical pressure during the filling phase that facilitates the transport of urine across the VUJ [33]. The abnormal bladder with increased intravesical pressure can impair ureteral emptying. Therefore, the initial ureteric response is by increasing its peristaltic frequency [34]. After a certain point, the ureter starts to dilate and urinary stasis occurs. The intravesical pressure at which the ureter may start to decompensate is about 40 cm H2O.
The VUJ does not relax to allow the passage of urine [35]. It rather maintains an antireflux mechanism and facilitates the passage of the urine bolus by the sliding action of the distal ureter within its sheath as the bolus of urine is ejected into the bladder [36]. This mechanism of telescoping of the ureter within its sheaths decreases the VUJ resistance to flow and facilitates urine passage into the bladder. Various factors may affect the transfer of urine across the VUJ, which can be obstruction at the UVJ, raised intravesical pressure, or during very high flow rates that may exceed the transport capacity of the normal UVJ.
6.8 Whitaker Test
The Whitaker test is urodynamic study in which a contrast media is directly injected into the renal pelvis to delineate the anatomy while simultaneously monitoring renal pelvic and bladder pressures. It is used to differentiate a real obstruction form a permanent change in musculature of the upper urinary tract. The test can be also applied in postoperative patients to confirm the improvement in flow aided with an excretory urogram before urinary diversion is removed [37].
The classic technique is carried out by running water or saline into the ureter at a rate calculated to be greater than the maximum urine flow in diuresis, while at the same time the pressure is measured in the system (Figure 6.5). This suffers from the disadvantage that it is necessary to pass a fine needle and cannula into the dilated renal pelvis.

Figure 6.5 Whitaker test. Saline or water is infused into the renal pelvis through a percutaneous cannula while the pressure is monitored.
Pressure difference between the renal pelvis and bladder are measured as follows:
>22 mm H2O: Obstructed
18–22 mm H2O: Equivocal
<18 mm H2O: Non‐obstructed
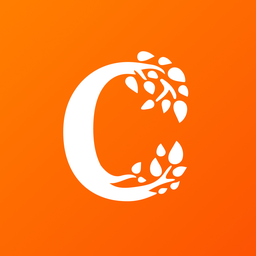
Full access? Get Clinical Tree
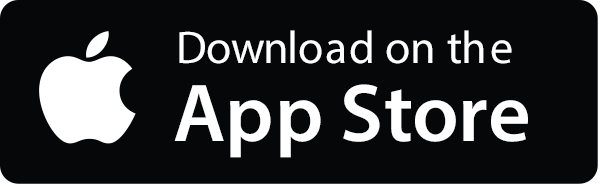
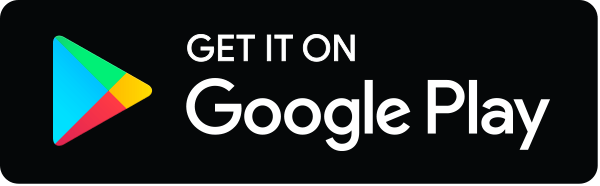