Outline
Introduction 203
Mechanisms of Kidney Development 204
Animal Cap in Fertilized Eggs of Amphibians 205
Embryonic Stem Cells 206
Creation of Induced Pluripotent Stem Cells 206
Cells and Animals to Use for the Generation of Induced Pluripotent Stem Cells 207
Mechanistic Understanding of Reprogramming Processes 207
Improving Methods for Induced Pluripotent Stem Cell Induction 208
Increasing Efficiency of Induced Pluripotent Stem Cell Induction 209
Complete Pluripotency 210
Disease Modeling with Patient-derived Induced Pluripotent Stem Cells 210
Toxicology Screening Using Induced Pluripotent Stem Cells and Embryonic Stem Cells 210
Future Directions in Induced Pluripotent Stem Cell Research and Technology 210
Cues to Regenerate Kidney Lineage Cells from Induced Pluripotent Stem Cells and Embryonic Stem Cells 211
Conclusion 212
Acknowledgment 213
Renal transplantation has proven a successful treatment for patients with end-stage renal failure. However, the therapy is hampered by the serious shortage of donor organs. Regenerative medical strategies using stem cells, including cell transplantation therapy, need to be developed to solve the problem. Recent advances in nuclear reprogramming technology allow for the transformation of terminally differentiated, adult cells into induced pluripotent stem (iPS) cells whose phenotype is indistinguishable from embryonic stem cells. This leap forward enables the creation of patient-specific pluripotent cell lines that carry disease genotypes. These pluripotent stem cell lines could be used both as in vitro models for the study of disease and as potential sources of material for cell replacement therapy. This chapter reviews the current status of research on the directed differentiation of pluripotent stem cells into renal lineages, and also describes recent advances and future directions of the iPS cell research and technology.
Introduction
An increasing number of patients with end-stage renal failure are undergoing dialysis therapy worldwide. It causes both medical and medicoeconomic problems. Renal transplantation has proven a successful therapy for most patients with end-stage renal failure, as the therapy results in a significant improvement in the patient’s quality of life, prolongs survival and is considered cost-effective . However, the annual increase in the number of new patients with end-stage renal disease who need a renal transplant, and the widening gap between the demand for and the supply of donor kidneys have led to a progressive shortage of donor organs for transplant. This has become a serious issue and is worsened by the problem of limited graft survival due to immune rejection .
Among the strategies to overcome these problems is kidney regeneration using stem cells. Stem cells may be divided into two large categories: organ-specific or somatic stem cells and pluripotent stem cells. In contrast to organ-specific stem cells that generally have a limited potential for growth and differentiation, pluripotent stem cells, such as embryonic stem cells (ESCs) and induced pluripotent stem (iPS) cells , have a virtually unlimited replicative capacity on culture dishes and are theoretically able to give rise to any cell type in the body. Stem cells have increasingly been used as a model system for understanding developmental mechanisms. In addition, in vitro culture and differentiation of stem cells offer unique opportunities for disease modeling, drug discovery, toxicology and cell replacement therapy . The generation of specific functional cell types from ESCs has been demonstrated, including neural cells (several kinds of neuron and glia), vascular endothelia and smooth muscle, cardiomyocytes, hematopoietic cells, pancreatic insulin-producing cells and hepatocyte-like cells . However, the protocol for in vitro differentiation of pluripotent stem cells into renal lineage cells has not been fully established.
Other approaches to regenerate kidney have also been investigated using organ-specific local stem cells within the kidney and bone marrow-derived hematopoietic stem cells . Kidney regeneration using mesenchymal stem cells localized in bone marrow has also been examined . However, the approaches are still being developed and the role of these stem cells in kidney regeneration remains to be well defined.
Therapeutic approaches using human ESCs face two major problems. One is the ethical issue derived from the use of human fertilized eggs, and the other is immune rejection in any cell or tissue transplantation due to histocompatibility antigenic differences between ESCs and patients. These problems have been overcome by a breakthrough experiment by Takahashi and Yamanaka. They identified four factors normally found in ESCs, Oct3/4, Sox2, c-Myc and Klf4, that were sufficient to reprogram both mouse and human somatic cells to closely resemble mouse and human ESCs . They named these iPS cells. Since iPS cells can be generated from somatic cells of patients, clinical approaches using iPS cells are not associated with the two above problems (use of human fertilized egg and immune rejection). In the next natural step after iPS cell creation, significant progress has been made in redifferentiating iPS cells into somatic cells. As is the case with ESCs, iPS cells have been redifferentiated into several somatic tissues, including active motor neurons , insulin-secreting islet-like clusters , hepatocyte-like cells and a number of cardiovascular cells (arterial endothelium, venous endothelium, lymphatic endothelium, cardiomyocytes), but not kidney .
This chapter first summarizes the mechanisms of kidney development and the research on the directed differentiation of ESCs into renal lineages based on the knowledge of kidney development. In vitro generation of kidney using the undifferentiated cell mass in amphibian eggs, similar to mammalian pluripotent stem cells in that the cell mass can differentiate into various organs in vitro, is also described as a reference to kidney regeneration in mammals. Recent advances in the iPS cell research and technology are then reviewed, and finally the future direction of iPS cells in the field of regenerative nephrology is described.
Mechanisms of Kidney Development
Vertebrates develop successively three kidneys: pronephros, mesonephros and metanephros. The three kidneys consist of a basic functional unit, the nephron, although the number of nephrons differs among kidneys . The kidneys are derived from a portion of the early embryonic germ layer, the intermediate mesoderm, that is located between the lateral and paraxial mesoderms . A lineage tracing experiment has demonstrated that intermediate mesodermal cells expressing Odd-skipped related ( Osr )- 1 , an essential transcriptional factor for intermediate mesoderm and kidney development, give rise to all components consisting of mammalian adult kidney metanephros . Metanephros is formed by the reciprocal interaction between two tissues derived from the intermediate mesoderm: the metanephric mesenchyme and the ureteric bud . The ureteric bud induces the metanephric mesenchyme to differentiate into the epithelia of glomeruli and renal tubules. By creating a novel culture system which uses the coculture with the cell line expressing a renal-epithelializing factor Wnt4 , it was demonstrated that mouse metanephric mesenchyme contains multipotent progenitor cells that can give rise to several kinds of epithelial cells found in adult kidney, such as glomerular podocytes and epithelia of proximal and distal renal tubules and the loop of Henle . The progenitor cells are contained only in the cell population strongly expressing Sall1 , a zinc finger transcriptional factor that is essential for kidney development. It was also shown that the progenitor cell population can reconstitute a three-dimensional kidney structure in vitro, which contains glomerulus- and tubule-like components in an organ culture setting ( Fig. 13.1 A–C ). The presence of progenitor cells was also demonstrated in vivo using a lineage tracing experiment for Six2 , which is also an essential transcriptional factor for the formation of kidney .

The progenitors in the metanephric mesenchyme differentiate into epithelia after Wnt stimulation, but a cell fate decision is required for further differentiation towards glomerular podocytes, proximal or distal renal tubules, or the loop of Henle. The molecular mechanisms of the cell fate decision are largely unknown, although it was demonstrated that Notch2 is required for the differentiation of proximal nephron structures, such as podocytes and proximal renal tubules, as Notch2 deletion leads to the impaired formation of these proximal structures . There may be many other molecules involved in the lineage commitment, and they remain to be elucidated. Metanephric mesenchyme may contain at least two other precursor populations in addition to the epithelial progenitors: (i) vascular precursor cells that can give rise to vascular and glomerular endothelial cells, vascular smooth muscle cells (pericytes) and mesangial cells, and (ii) stromal precursors eventually differentiating into interstitial cells within the adult kidney. The ureteric bud is known to elaborate the lower urinary tract system, from collecting ducts through renal pelvis and ureters to a part of the urinary bladder . Most of the mechanisms of the lineage commitment, by which the intermediate mesoderm gives rise to the ureteric bud, stromal and vascular cells, are unknown. Further investigations are required to elucidate the mechanisms, which will eventually help us to completely understand the commitment of multiple cell lineages within a kidney and reproduce it in vitro from pluripotent stem cells.
Mechanisms of Kidney Development
Vertebrates develop successively three kidneys: pronephros, mesonephros and metanephros. The three kidneys consist of a basic functional unit, the nephron, although the number of nephrons differs among kidneys . The kidneys are derived from a portion of the early embryonic germ layer, the intermediate mesoderm, that is located between the lateral and paraxial mesoderms . A lineage tracing experiment has demonstrated that intermediate mesodermal cells expressing Odd-skipped related ( Osr )- 1 , an essential transcriptional factor for intermediate mesoderm and kidney development, give rise to all components consisting of mammalian adult kidney metanephros . Metanephros is formed by the reciprocal interaction between two tissues derived from the intermediate mesoderm: the metanephric mesenchyme and the ureteric bud . The ureteric bud induces the metanephric mesenchyme to differentiate into the epithelia of glomeruli and renal tubules. By creating a novel culture system which uses the coculture with the cell line expressing a renal-epithelializing factor Wnt4 , it was demonstrated that mouse metanephric mesenchyme contains multipotent progenitor cells that can give rise to several kinds of epithelial cells found in adult kidney, such as glomerular podocytes and epithelia of proximal and distal renal tubules and the loop of Henle . The progenitor cells are contained only in the cell population strongly expressing Sall1 , a zinc finger transcriptional factor that is essential for kidney development. It was also shown that the progenitor cell population can reconstitute a three-dimensional kidney structure in vitro, which contains glomerulus- and tubule-like components in an organ culture setting ( Fig. 13.1 A–C ). The presence of progenitor cells was also demonstrated in vivo using a lineage tracing experiment for Six2 , which is also an essential transcriptional factor for the formation of kidney .

The progenitors in the metanephric mesenchyme differentiate into epithelia after Wnt stimulation, but a cell fate decision is required for further differentiation towards glomerular podocytes, proximal or distal renal tubules, or the loop of Henle. The molecular mechanisms of the cell fate decision are largely unknown, although it was demonstrated that Notch2 is required for the differentiation of proximal nephron structures, such as podocytes and proximal renal tubules, as Notch2 deletion leads to the impaired formation of these proximal structures . There may be many other molecules involved in the lineage commitment, and they remain to be elucidated. Metanephric mesenchyme may contain at least two other precursor populations in addition to the epithelial progenitors: (i) vascular precursor cells that can give rise to vascular and glomerular endothelial cells, vascular smooth muscle cells (pericytes) and mesangial cells, and (ii) stromal precursors eventually differentiating into interstitial cells within the adult kidney. The ureteric bud is known to elaborate the lower urinary tract system, from collecting ducts through renal pelvis and ureters to a part of the urinary bladder . Most of the mechanisms of the lineage commitment, by which the intermediate mesoderm gives rise to the ureteric bud, stromal and vascular cells, are unknown. Further investigations are required to elucidate the mechanisms, which will eventually help us to completely understand the commitment of multiple cell lineages within a kidney and reproduce it in vitro from pluripotent stem cells.
Animal Cap in Fertilized Eggs of Amphibians
Amphibians have been used as experimental animals for research into developmental biology mainly because of the ease with which they can be handled and observed. In amphibian eggs, the ectodermal cell mass of mid-blastula embryos, called the animal cap, is similar to ESCs and iPS cells in mammals in that it possesses a potential of multipotent differentiation. The animal cap can be easily excised from fertilized eggs and cultured as explants in vitro in simple saline solution. After treatment with differentiation-inducing factors, the animal cap can differentiate into various tissues and organs in vitro . The pronephros is a simple excretory organ of Xenopus larvae with only one nephron, consisting of the glomus, a filtering unit equivalent to the glomerulus in metanephros, tubule and duct. When animal caps were treated with a combination of activin A, a protein inducer belonging to transforming growth factor-β (TGF-β) superfamily, and retinoic acid, differentiation into pronephric tubules was observed . In the same differentiation system, the marker gene expression for pronephric glomus was also detected . It was demonstrated that the pronephric duct, a third component of the pronephric kidney, can be generated in the explants, in addition to the pronephric tubule and glomus, and that the pronephros formed in vitro is similar to that in Xenopus embryos both histologically and in gene expression ( Fig. 13.1 D–F). The pronephric tubule and duct also show similar ultrastructure to those of Xenopus embryos by electron microscopy.
The pronephros formed in vitro from the animal cap shows a temporarily similar gene expression pattern to that in Xenopus embryos. Using the in vitro system to generate pronephric tissue, the molecular mechanisms underlying pronephros development have been investigated and some molecules involved in the formation of the pronephros were identified . Although the pronephros produced in vitro from the animal cap cannot be directly translated to clinical applications, this system should facilitate the study of kidney regeneration and may promote a shift from tissue engineering to clinical applications. Indeed, this induction method established for the animal cap using activin A and retinoic acid has been applied in a differentiation experiment from mouse ESCs into renal lineages .
Embryonic Stem Cells
ESCs are pluripotent stem cells derived from the inner cell mass of fertilized eggs in mammals. The first derivation of mouse ESCs by Evans and Martin was described in 1981 and human ESCs was established by Thomson about 20 years later . Both mouse and human ESCs have a virtually unlimited replicative capacity and are theoretically able to give rise to any cell type in the body. Since their derivation, mouse ESCs have mainly been used as a research tool to generate experimental mouse models by combination with gene targeting techniques. However, following successes in human ESC derivation, the regenerative medical strategy has been considered closer to clinical applications, which aim at accomplishing the functional recovery of dysfunctional organs by transplanting organ-specific cell types generated from ESCs in vitro. The in vitro differentiation of ESCs into specific cell types of various organs has since been more vigorously investigated to develop cell transplantation therapies.
In vivo injection of ESCs into immunocompromised mice can produce teratoma, which are tumors containing cells of all three embryonic germ layers (ectoderm, mesoderm and endoderm). Glomerulus- and tubule-like structures can be formed in teratomas derived from mouse and human ESCs , which demonstrates that mouse and human ESCs do have a potential to differentiate into kidney lineage cells. Furthermore, fundamental research aiming to establish methods to efficiently induce mouse ESCs into renal progenitors and fully differentiated renal cell types have already been carried out . Mouse ESCs treated with the combination of hepatocyte growth factor (HGF) and activin A in addition to the transfection of a renal-epithelializing factor Wnt4 , the combination of activin A, bone morphogenetic protein (BMP)-7 and retinoic acid , activin A alone , BMP-4 alone , or the combination of four chemical compounds produced cells expressing markers for intermediate mesoderm, developing kidney and fully differentiated renal cells. In some reports, the induced cells formed tubule-like structures or were incorporated into developing mouse kidneys . Few reports have described the directed differentiation of human ESCs into a renal lineage. It was shown that human ESCs differentiated in vitro into cells expressing WT1 and rennin, marker genes for glomeruli, following treatment with growth factors . Combinational treatment with activin A, retinoic acid and BMP-4 or BMP-7 also induces marker gene expression for intermediate mesoderm and developing kidney from human ESCs .
In summary, these data suggest that ESCs are a potential source for kidney regeneration, although the efficiency of generation and the induction rate of renal lineage cells from ESCs are unknown in many cases. It remains to be determined whether renal lineage cells produced from ESCs have excretory or endocrinological functions as in the kidney. It is also unknown whether tubule-like structures or cells integrated into the kidney are metanephric or of earlier kidneys (pronephros and mesonephros). The research on kidney regeneration using ESCs is in its infancy and further studies are required to develop this research field.
Creation of Induced Pluripotent Stem Cells
Development involves two distinct cellular processes: division and differentiation. Division is the means by which one cell gives rise to two daughter cells, and is indispensable for the growth of an organism and the renewal of fully developed tissues. Differentiation refers to the process by which a cell specializes to perform a particular biological function in an adult. Differentiation usually occurs through a combination of cell–cell interactions, exposure to diffusible factors and other positional cues that ultimately alter gene expression, conferring a specific cellular identity and function.
One of the more remarkable observations made in the past century was that differentiation is not a unidirectional process. Instead, it can be turned back much like the hands of a clock. This rewinding of the developmental clock is termed nuclear reprogramming and is often defined as the process whereby an adult somatic nucleus has a developmental potential restored to it . Nuclear reprogramming has been accomplished in three ways: (i) somatic cell nuclear transfer (SCNT) or cloning, (ii) cell fusion, and (iii) factor-based reprogramming to produce iPS cells.
SCNT, a procedure in which the nucleus of an adult cell is injected into an unfertilized egg whose chromosomes have been removed, has demonstrated that the genome of adult cells can be reset to an embryonic state . Using this strategy researchers have generated cloned embryos that possess the potential to develop into an adult animal or become an ESC line that is genetically identical to that of the donor nucleus . These experiments established that no irreversible changes are made to the genome during development and further showed that animal oocytes harbored factors that could accomplish nuclear reprogramming.
In a related series of experiments, several researchers have shown that when somatic cells are fused with ESCs, the resulting tetraploid hybrid cells silence the expression of somatic genes and establish a program of transcription indistinguishable from ESCs, indicating that ESCs contain the necessary reprogramming activities to accomplish this transformation . Thus, the cytoplasm of the enucleated oocyte and the ESC is able to re-establish the pluripotent state via a mechanism dependent on global epigenetic and transcriptional changes.
The mechanism by which this transformation occurred and the mediators of nuclear reprogramming were largely undefined until Takahashi and Yamanaka identified four factors normally found in ESCs that could reprogram mouse somatic cells (see Introduction). They called these iPS cells and these stem cells were also derived from human somatic cells 1 year later ( Fig. 13.2 A, B ) . Since this first report, the technique has been rapidly confirmed, improved and subsequently applied to successfully reprogram somatic cells. This technology has since given birth to an entire research field that has progressed at a phenomenal pace.

Cells and Animals to Use for the Generation of Induced Pluripotent Stem Cells
The rate at which iPS cell lines are being created is rapidly increasing. While most iPS cells were created from fibroblasts in the beginning, cell lines have also been created from adult liver and stomach cells , adult neural stem cells , keratinocytes , hematopoietic cells , fetal cells harvested during both amniotic fluid and chorionic villus sampling, and several other somatic cell types . It appears that the reprogramming is possible in almost any cell type, although the efficiency of the reprogramming process varies among cell types. Finding the most suitable somatic cell types to accomplish reprogramming remains a main challenge for iPS cell research.
The derivation of iPS cell lines from species other than human or mouse cells expands the research potential of iPS cell in additional animal models. The generation of iPS cells was reported from adult rhesus monkey fibroblasts . Two separate groups also created iPS cells from adult rat cells .
In addition, iPS cells have been derived from somatic pig cells by two other groups. One group used tetracycline-inducible human Oct4/Sox2/Klf4/c-Myc/Nanog/Lin28 delivered via lentiviruses to transduce primary pig ear fibroblasts and primary bone marrow cells into iPS cells . The other group transduced porcine fetal fibroblasts into iPS cells using human Oct4/Sox2/Klf4/c-Myc delivered with lentiviruses . It has been reported that iPS cells were generated from canine cells by the combination of retroviral transduction and chemical inhibitors . It is possible that culture conditions for the derivation or maintenance of iPS cells from species other than human or mouse have not been fully established. However, the availability of model animal iPS cells offers a new and potentially powerful model for therapeutic applications.
Mechanistic Understanding of Reprogramming Processes
One of the major questions in iPS cell research seeks to define the underlying mechanism by which nuclear reprogramming is accomplished. This includes identifying the molecular players, the key cellular and molecular events and the likely ways in which this process might fail. The goal is to make the iPS induction process safer and more efficient, and one day to manipulate the underlying cellular state of any cell, thereby generating specific cell types at will. Indeed, it was demonstrated that in vivo expression of the combination of three defined factors, Ngn3 (also known as Neurog3), Pdx1 and Mafa, which are key developmental regulators of the pancreas, can reprogram differentiated exocrine cells in adult mice into cells that closely resemble beta-cells . It has also been shown that the combination of three factors, Ascl1, Brn2 (also known as Pou3f2) and Myt1l, rapidly and efficiently converted mouse embryonic and postnatal fibroblasts into functional neurons in vitro . The neurons generated were named induced neuronal (iN) cells.
The nature of the factors required for the reprogramming of somatic cells into iPS cells has been elucidated. In particular, Oct4 has emerged as a central molecule in iPS cell reprogramming; for example, neural stem cells can be reprogrammed to iPS cells with Oct4 alone, which was not unexpected as they express high levels of endogenous Sox2 . Until recently, no cell line had been reprogrammed without Oct4. However, it has been shown that the orphan nuclear receptor Nr5a2 (also known as Lrh-1) can replace Oct4 in the derivation of iPS cells from mouse somatic cells in part through activating Nanog expression . While Yamanaka’s original four-factor combination remains the most widely used, several other combinations have been shown to generate iPS cells. One of the earliest alternative combinations was developed by the Thomson group. They successfully used Oct4/Sox2/Nanog/Lin28 to create iPS cell lines . Recently, it has been shown that mouse embryonic fibroblasts (MEFs) were able to reprogram using Esrrb, which is an orphan nuclear receptor, in combination with Oct4, Sox2 and c-Myc .
Several reports have shown that some of the reprogramming factors can be replaced with chemicals. In these cases, chemicals either directly activate the expression of reprogramming factors or in some way compensate for their activity. A Harvard group added valproic acid (VPA), known to be a histone deacetylase (HDAC) inhibitor and a widely used antiepileptic drug, to newborn human skin (fibroblast) cells in culture and was able to create iPS cells with only two reprogramming factors, Oct4 and Sox2 , eliminating the need for two potent cancer-promoting genes, c-Myc and Klf4 . Another group used a combination of the small molecules BIX-01294 and BayK8644 to generate iPS cells from MEFs that were transfected with only Oct4 and Klf4 . It has also been demonstrated that a small-molecule inhibitor of TGF-β signaling can replace Sox2 by inducing Nanog expression . The promise of these approaches is to generate iPS cells using chemicals alone. However, great care should be taken over the toxicity of chemicals, including their carcinogenicity.
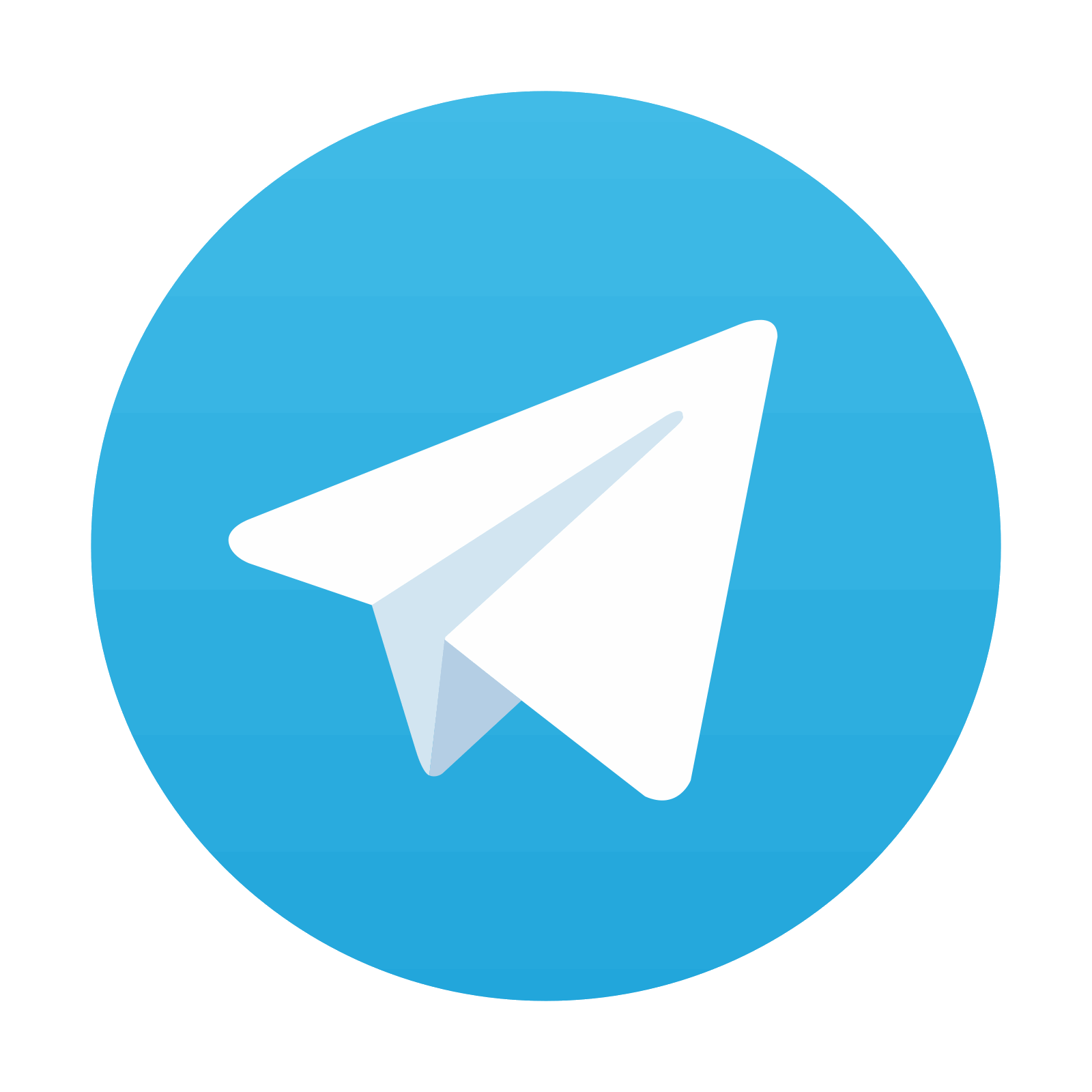
Stay updated, free articles. Join our Telegram channel
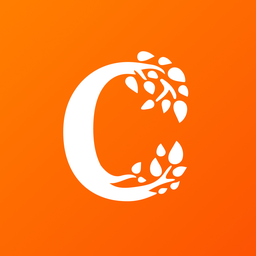
Full access? Get Clinical Tree
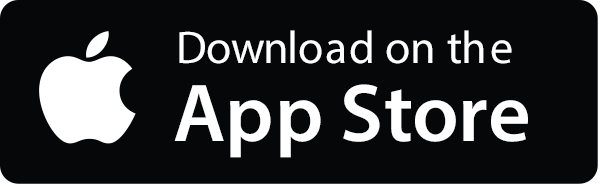
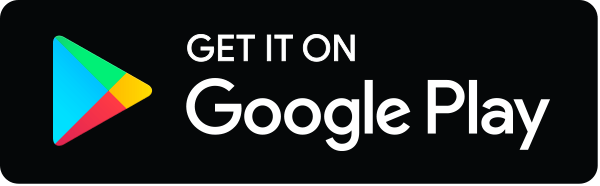