Abstract
Patients with chronic kidney disease (CKD) are very complex with regard to disease, comorbidity, number of medications, and dosage regimens. In this chapter we discuss (1) the implications of creatinine standardization and use of estimated creatinine clearance or glomerular filtration rate in drug dosing assessment in CKD patients and in special CKD populations (obese and elderly); (2) key principles that all clinicians should be aware of when prescribing medications for patients with CKD; (3) pharmacokinetic and dosing information on selected newer drugs (direct oral anticoagulants, newer diabetes agents); (4) how to assess a new drug for dialyzability and initial dosing in a patient receiving kidney replacement therapy (KRT); and (5) evidence to suggest best practices for team care, transitions of care, and informatics approaches or tools that may improve health outcomes and reduce costs.
Keywords
chronic kidney disease, diabetes, estimating equations, medication dosing, pharmacokinetics, medication dialyzability, transition of care, team care
Outline
Assessment of Kidney Function for Drug Dosing, Including Special Populations, 251
General Pharmacokinetic and Pharmacodynamic Principles, 253
General Approach for Drug Regimen Design in Chronic Kidney Disease, 256
Dosing of Select Newer Agents in Chronic Kidney Disease, 257
Direct Oral Anticoagulants, 257
Agents for Type 2 Diabetes Mellitus—Sodium–Glucose Cotransporter 2 Inhibitors, 259
Agents for Type 2 Diabetes Mellitus—Dipeptidyl Peptidase-4 Inhibitors, 264
Agents for Type 2 Diabetes Mellitus—Glucagon-Like Peptide-1 Receptor Agonists, 264
Metformin, 265
Drug Dosing in Dialysis Patients, 265
Considerations For Drug Removal by Renal Replacement Therapies, 266
Case Example: Dosing Brivaracetam in Hemodialysis and Continuous Kidney Replacement Therapy, 267
Drug Interactions In Chronic Kidney Disease, 269
Importance Of Interdisciplinary Teams in Improving Chronic Kidney Disease Care, 269
Improving Chronic Kidney Disease Care During Transitions, 270
Informatics Approaches to Improve Chronic Kidney Disease Care, 270
Assuring safe, effective, and convenient drug therapy for patients with chronic kidney disease (CKD) is challenging from multiple perspectives. Many drugs are partially or wholly excreted by the kidneys; these drugs are typically dose-reduced to produce similar blood or plasma concentrations as in patients with normal kidney function. Often, compendia such as Micromedex, AHFS, and Lexicomp recommend dosing based on categories of creatinine clearance (CrCL) or glomerular filtration rate (GFR). In the United States, most creatinine assays used by US clinics or hospitals have been calibrated to an isotope dilution mass spectrometry (IDMS) reference (creatinine standardization) so results are consistent across laboratories. However, this creates a dilemma for dosing adjustment. The pharmacokinetics of most marketed US drugs that require dosage adjustment in CKD patients have been evaluated using the Cockcroft–Gault (CG) estimated CrCL (eCrCL) formula (including patient weight), which was developed before creatinine standardization. However, most clinics and hospitals automatically report estimated GFR (eGFR) normalized to body surface area (BSA) with each serum creatinine level, that clinicians use to determine drug dosage adjustments. We discuss the implications of creatinine standardization and use of eCrCL or eGFR in drug dosing assessment in CKD patients and in special CKD populations (obese and elderly).
Kidney dysfunction decreases elimination of drugs that are mainly cleared by the kidney, but kidney dysfunction can also affect drug absorption, distribution, and metabolism, as well as drug targets or effector proteins. Thus kidney dysfunction can greatly alter drug pharmacokinetics and pharmacodynamics, causing variable drug efficacy and toxicity in CKD patients compared with patients with normal kidney function. We discuss key principles that all clinicians should be aware of when prescribing medications for patients with CKD and present pharmacokinetic and dosing information on selected newer drugs (direct oral anticoagulants, newer diabetes agents).
Clinicians seeing maintenance dialysis patients face added complexities related to the effects of dialysis therapy when determining drug dosage adjustments. We discuss drug- and dialysis-related characteristics that predict dialyzability and where to find updated information on drug dialyzability, and we provide an example of how to assess a new drug for dialyzability and initial dosing in a patient receiving renal replacement therapy (RRT).
Patients with CKD are very complex with regard to disease, comorbidity, number of medications, and dosage regimens. Consequently, hospitalizations are frequent and healthcare costs are high. We review the evidence to suggest best practices for team care, transitions of care, and informatics approaches or tools that may improve health outcomes and reduce costs.
Assessment of Kidney Function for Drug Dosing, Including Special Populations
Assessment of kidney function is important for drug regimen design with drugs that depend on renal clearance. Although direct measurement of GFR using a marker such as iothalamate is most accurate, it is not practical in the clinical setting. Estimating equations include the CG, Modification of Diet in Renal Disease (MDRD), and Chronic Kidney Disease-Epidemiologic Prognosis Initiative (CKD-EPI) equations (with and without cystatin C). (See Chapter 2 for detailed information on measurement and estimation of kidney function.) The CG equation estimates CrCL, a surrogate marker of GFR. The MDRD and CKD-EPI equations were developed using measured iothalamate clearance, and they estimate GFR. The eGFR has become a standard laboratory result reported by most clinical laboratories when serum creatinine level is available; eGFR has been recommended for determining and classifying CKD and for drug dosing. The most recent Kidney Disease: Improving Global Outcomes (KDIGO) CKD guideline recommends the CKD-EPI equation as the eGFR method for routine clinical practice. As it replaces the MDRD equation, clinicians should consider its specific performance for drug dosing.
Regardless of the method used, the limitations of these estimates for drug dosing must be considered. Creatinine standardization began in 2005 and was fully implemented by most US laboratories by 2010. Before assay standardization, serum creatinine values were overestimated by approximately 10% to 20%. Standardization led to less variability in creatinine results; however, only newer equations such as the reexpressed MDRD equation, CKD-EPI equations, and Schwartz equation for children, use standardized creatinine. For many drugs, manufacturer dosing recommendations are based on eCrCL using the CG equation, as this was the method used to assess kidney function in the pharmacokinetic studies. As many of these studies were performed before creatinine standardization, measured serum creatinine values were, on average, higher (leading to lower eCrCL values). Although adjustment methods have been suggested, valid reexpression of the CG equation is not possible, as plasma creatinine samples used to develop it are no longer available to be assayed by a standardized method. Controversially, the National Kidney Foundation recently recommended the CG equation only for research purposes, given that it cannot be reexpressed using standardized creatinine values and the potential for inaccurate results. At present, however, not enough data support replacing the CG equation exclusively with eGFR methods, and the CG equation remains widely used in clinical practice for drug dosing. The eGFR has been recommended for use in pharmacokinetic studies for new drugs being developed. The US Food and Drug Administration (FDA) 2010 draft of the Guidance for Industry related to pharmacokinetic studies in patients with impaired renal function recommended that pharmacokinetic studies be conducted using both eGFR and eCrCL. If such studies become standard and new evidence regarding drug dosing with eGFR becomes available, support may increase for use of eGFR for drug dosing in clinical practice.
A key study that led to the recommendation to use eGFR for drug dosing compared differences in dosing recommendations based on FDA-approved dosing for 15 drugs eliminated by the kidney. Discrepancies in recommended doses using measured GFR were compared with recommendations using MDRD eGFR and CG eCrCL using total and ideal body weight (TBW, IBW) for 5504 individuals. Agreement was highest for the MDRD eGFR (88%), followed by the CG eCrCL TBW (85%) and the CG eCrCL IBW (82%). Of note, one controversy about this study relates to dosing recommendations for the drugs evaluated being based on measured GFR, not measured or estimated CrCL, which was the method used by the manufacturers in the initial pharmacokinetic studies. Several other studies have compared dosing recommendations based on estimates using eGFR and CG eCrCL; however, they vary with regard to the equations used and the reference method for dosing comparison. Most discrepancies occur in patients at extremes of body weight and the elderly. For most of these studies, the clinical significance has not been determined, but discrepancies clearly exist. Findings such as these have led practitioners to question whether these estimates can be used interchangeably for drug dosing.
Discrepancies in dosing recommendations for obese patients using eGFR versus eCrCL highlight body size as an important factor to consider with current assessment equations for drug dosing. The MDRD and CKD-EPI equations, when developed, were normalized to a BSA of 1.73 m 2 , and their values are reported in mL/min/1.73 m 2 . This differs from the CG eCrCL, which is reported in mL/min. In general, when using the MDRD or CKD-EPI equation for drug dosing, the result should be multiplied by the patient’s BSA divided by 1.73 m 2 to convert the values to mL/min. This is particularly important for underweight or overweight individuals whose BSA differs substantially from 1.73 m 2 . With regard to body size, the weight to use in the CG equation is somewhat controversial. Creatinine is a metabolic by-product of muscle metabolism derived from creatine, and its production and release are affected by muscle mass, not fat, which makes use of TBW in overweight/obese individuals a less-reliable body size descriptor in the CG equation. Other body size descriptors that have been used include IBW, lean body weight (LBW), or an adjusted body weight (ABW). Using LBW in the CG equation provided the most unbiased, precise, and accurate estimate of measured CrCL in 54 morbidly obese individuals. However, based on the performance of ABW in the CG equation compared with measured CrCL in over 2800 individuals with body mass index (BMI) ≥25 kg/m 2 , use of the “TIA” method was recommended: use T BW in the CG equation when BMI is <18.5 kg/m 2 (underweight), I BW when BMI is 18.6 to 24.9 kg/m 2 , and A BW when BMI is ≥25 kg/m 2 ( Table 17.1 ). Of note, adjustment of eGFR for BSA has been shown to overestimate kidney function in morbidly obese (BMI >40 kg/m 2 ) patients ; the CG equation with the TIA method may provide a more reasonable estimate for drug dosing for such patients.
Weight Descriptor | Calculation |
---|---|
ABW (kg) | IBW + 0.4 (TBW − IBW) |
BMI (kg/m 2 ) | Weight/height 2 |
IBW for males (kg) | 50 + 2.3 kg (for every inch over 5 feet) |
IBW for females (kg) | 45.5 + 2.3 kg (for every inch over 5 feet) |
TBW (kg) | Actual weight in kg |
Variations in drug dosing recommendations are also notable in elderly patients, emphasizing the importance of considering age. Unfortunately, when available estimating equations were developed, relatively few elderly individuals were included. The CG equation was developed using data from 249 predominantly male veterans with 45% aged 60 years or older (oldest, 92 years). The MDRD equation was developed using data from 1070 individuals with a mean age of 51 ± 13 years and 22% aged older than 65 years. The CKD-EPI population included 5504 individuals of whom 15% were aged older than 65 years, and the CKD-EPI creatinine-cystatin C 2012 population included 5352 individuals of whom 13% were older than 65 years. More variation among subgroups based on age occurred in the MDRD study in individuals with eGFR ≥60 mL/min/1.73 m 2 . At these higher eGFR levels, eGFR overestimated measured GFR in individuals aged older than 65 years. In subsequent analysis of the MDRD and CKD-EPI equations, the CKD-EPI equation performed similarly to the MDRD equation in individuals aged older than 65 years. The challenges with using estimating equations for drug dosing in elderly patients relate to whether differences in the eGFR and CG with increasing age are recognized. In the mathematical simulation study described by Delanaye et al., CG eCrCl results were progressively lower than CKD-EPI eGFR results with increasing age. After age 60 years, CKD-EPI results began to exceed CG results, and by age 95 years, the CKD-EPI result was 40% higher than the CG result. The Berlin Initiative Study equation has been proposed as a better estimate of kidney function in the elderly ; however, this equation showed no significant advantage over the CKD-EPI creatinine-cystatin C equation for estimating gentamicin clearance in 99 individuals older than 70 years. The European Renal Best Practice Group noted the limitations of using the available estimating equations in elderly patients with eGFR <45 mL/min/1.73 m 2 and risk for kidney function misclassification and recommends measuring GFR or using the CKD-EPI equation with both creatinine and cystatin C when more precise estimation is needed. Because serum creatinine becomes less reliable as a marker of kidney function as muscle mass declines (as in the elderly), some practitioners suggest that serum creatinine be rounded up to 1.0 in elderly individuals with a measured creatinine <1 to avoid overestimating kidney function. However, this practice has not been shown to improve the performance of the estimate and is not recommended.
Clearly, choice of body size descriptor (i.e., TBW, IBW, ABW) in the CG equation and individualizing the eGFR estimate for BSA (i.e., multiplying by BSA/1.73 m 2 ) has a substantial effect on estimates of kidney function. Table 17.2 shows an example of the differences in estimates based on the method used. In scenario 1, the individual is close to IBW; therefore the weight used in the CG equation has little effect on the results and his eCrCL is approximately 40 mL/min. The CKD-EPI eGFR without adjustment for BSA is 27 mL/min/1.73 m 2 , but 35 mL/min when adjusted for BSA. Although the difference in adjusted and unadjusted CKD-EPI results is relatively minor, these estimates straddle a typical dosing threshold of 30 mL/min. The dosing decision for a drug requiring adjustment or discontinuation with a GFR or CrCL <30 (e.g., enoxaparin, metformin, dabigatran) would become more challenging. In this case, the adjusted value of 35 mL/min is more appropriate. This example highlights the similarity of the CKD-EPI estimate in mL/min and the CG eCrCL estimate, and drug dosing recommendations would not differ based on method because the estimates are 30 to 40 mL/min. In scenario 2, the individual is obese. Now the eCrCL ranges from 41 mL/min with IBW to almost 60 mL/min with TBW. ABW would give a result close to 40 mL/min. The CKD-EPI unadjusted eGFR remains 27 mL/min/1.73 m 2 as in the first scenario, but adjusting for BSA yields a value of 40 mL/min. If the CKD-EPI equation is not adjusted for BSA, a drug with a dosing threshold at 30 mL/min could be underdosed. It could be overdosed by using the eCrCL with TBW, as results of 59 mL/min may be above another common dosing threshold of 50 mL/min.
Scenario | CG-TBW (mL/min) | CG-IBW (mL/min) | CG-ABW (mL/min) | CKD-EPI (mL/min/1.73 m 2 ) | CKD-EPI Adjusted (mL/min) |
---|---|---|---|---|---|
58-year-old white male; SCr 2.5 mg/dL; Ht 76 in; Wt 90 kg; IBW = 87 kg; BSA 2.21 m 2 | 41 | 40 | 40 | 27 | 35 |
Scenario 1 with wt = 130 kg BSA = 2.58 | 59 | 41 | 47 | 27 | 41 |
90-year-old white female; SCr 1.4 mg/dL; Ht 65 in; Wt 52 kg; IBW = 57 kg; BSA 1.56 m 2 | 22 | ∗ | ∗ | 33 | 30 |
90-year-old black female; SCr 0.7 mg/dL; Ht 65 in; Wt 52 kg; IBW = 57 kg; BSA 1.56 m 2 | 44 | ∗ | ∗ | 76 | 69 |
∗ Not appropriate to use because the patient’s weight is below her IBW.
As an example of age-related differences, scenario 3 in Table 17.2 shows CG eCrCL and CKD-EPI eGFR for a 90-year-old white woman with serum creatinine 1.4 mg/dL who is below her IBW. The CKD-EPI adjusted eGFR is 30 mL/min; however, the eCrCL based on TBW is 22 mL/min. Scenario 4 is this same individual with serum creatinine 0.7 mg/dL. The difference in eCrCL and the adjusted CKD-EPI result is 25 mL/min, which has substantial implications with a dosing threshold at 50 to 60 mL/min. Whether the serum creatinine is a reliable marker in this 90-year-old individual with reduced muscle mass would have to be determined. In this case, a measured CrCL or GFR may be reasonable if the drug has a narrow therapeutic index. The general implications of scenarios 3 and 4 are that older individuals would be prescribed higher doses of a given drug using the eGFR result as opposed to the CG result. This has been shown in several studies evaluating hypothetical dosing differences for drugs eliminated by the kidney when the CG results (using varying body weights) were compared to eGFR.
Clinicians should be well aware of the potential difference in estimates of kidney function in populations that include overweight and underweight individuals and the elderly and should take into account the effect on drug dosing decisions. Careful consideration of the method used to estimate kidney function, the method used to develop dosing recommendations for the drug, and the risk–benefit profile is warranted when designing a drug regimen in patients with reduced kidney function. The limitations of applying estimating equations for the purpose of drug dosing in obese individuals or in elderly individuals with reduced muscle mass are acknowledged by KDIGO and the National Kidney Disease Education Program (NKDEP). NKDEP states that “neither eGFR nor creatinine clearance will be accurate in individuals with extremes of body size or muscle mass . . . the limitations in creatinine-based estimating equations are particularly relevant for populations with reduced muscle mass, including the frail and elderly.”
Fortunately, more objective data are becoming available that support eGFR-based equations. Examples include vancomycin and gentamicin. Some studies have shown that CKD-EPI equations incorporating both creatinine and cystatin C (e.g., the CKD-EPI cr-cys C equation) are better predictors of drug clearance than other estimates. As additional evidence to support eGFR equation results for drug dosing becomes available and manufacturers incorporate eGFR into pharmacokinetic studies, practitioners may feel more comfortable with eGFR over eCrCL for assessing drug dosing. Until then, clinical judgment is important, considering the implications of overdosing or underdosing the specific drug in question. For example, if a drug eliminated by the kidney with a narrow therapeutic index or potential for serious toxicity (e.g., enoxaparin) is prescribed to an elderly individual, a measured CrCL or GFR may be warranted if therapeutic drug monitoring with plasma levels is not available. The method used when dosing recommendations were developed should also be considered. The package labeling for the recently approved direct oral anticoagulant (DOAC) betrixaban includes dosing adjustments for individuals with reduced kidney function, but specifically states that the method used to derive dosing recommendations was the CG equation with TBW. Since this drug was approved by the FDA in 2017, standardized creatinine was likely used to derive CG results. In this case, the CG equation with TBW should be used to estimate kidney function until newer evidence suggests otherwise. Clinical monitoring for desired effects and avoidance of adverse effects for any drug, particularly those with narrow therapeutic indices such as DOACs (e.g., apixaban, rivaroxaban), is essential to prevent medication-related problems in patients with CKD.
General Pharmacokinetic and Pharmacodynamic Principles
Pharmacokinetics describes the disposition of a drug in the body and is affected by a drug’s absorption, distribution, metabolism, and elimination (often referred to as ADME ), whereas pharmacodynamics describes the physiological effect of a drug. The effect of a drug is typically related to its concentration at the site of action. Although ideally the concentration would be measured at the receptor site, this is not practical. Therefore, the blood or plasma concentration of a drug is used as a surrogate to predict activity at the site of action for drugs with good correlation between the pharmacological response and the plasma concentration. In clinical practice, plasma concentration is generally measured for drugs with a narrow therapeutic index, meaning there is a narrow margin between a therapeutic and a toxic concentration, and for drugs for which the desired effect cannot be measured by any other practical method (e.g., hemoglobin response with epoetin alfa). For example, the concentration of phenytoin at its site of action in the central nervous system cannot be measured to make clinical decisions. Instead, plasma concentrations are measured with the goal of sustaining a concentration within the therapeutic range that correlates with the desired tissue concentration (e.g., free [unbound] phenytoin concentration 1 to 2 mg/L). Achievement of these concentrations at steady state (when the rate of drug input equals the rate of drug metabolism and/or elimination) depends on the pharmacokinetic characteristics or the ADME. In individuals with kidney disease, pharmacokinetic parameters are most often altered, as opposed to the pharmacodynamic effects. These parameters and the potential changes with CKD are reviewed here.
Absorption
Absorption is a process of drug movement from its site of delivery into the bloodstream. The bioavailability of a drug is the fraction of an administered dose that reaches the systemic circulation. The bioavailability for a drug administered intravenously is 100%, but is often less with oral administration. This is because oral drug absorption is affected by gastrointestinal (GI) transit time, edema of the GI tract, gastric pH, GI metabolism, and liver metabolism as the drug makes its first pass through the liver from the GI tract to reach systemic circulation. Metabolism occurs due to the activity of the cytochrome P450 enzymes in the GI tract and liver, which may be altered in individuals with kidney disease. Reduced activity of these enzymes can result in greater bioavailability of the parent drug or active metabolites, which may be clinically significant for drugs with a narrow therapeutic index that are extensively metabolized by these enzymes (e.g., cyclosporine). The activity of uptake and efflux transporters present in the GI tract, including P-glycoprotein (Pgp) and organic anion transporters (OATs), also affects bioavailability. Pgp on intestinal epithelial cells is responsible for efflux of certain drugs (e.g., apixaban, digoxin) and limits cellular uptake and absorption into enterocytes. These transporters are not necessarily changed in patients with CKD, but the patients may be taking drugs or other substances that are inhibitors (e.g., amiodarone, omeprazole, grapefruit juice), resulting in increased bioavailability, or inducers (e.g., St. John’s wort, phenytoin), resulting in decreased bioavailability. In addition, gastric pH may be altered by other drugs (e.g., proton pump inhibitors, H2-receptor antagonists) and high salivary urea concentrations (urea is converted to ammonia). Delayed gastric emptying due to gastroparesis in patients with diabetes and CKD may also delay the time to reach a maximum plasma concentration after orally ingesting a drug.
Distribution
The extent to which a drug distributes to body fluids and tissue is indicated by the volume of distribution (Vd), often expressed in L or L/kg. This is a theoretical volume necessary to account for the total amount of drug if it were present throughout the body at the same concentration as in the plasma. The Vd depends on the drug characteristics, including plasma protein binding (only unbound drugs may distribute extravascularly), total body water, and tissue binding. The plasma compartment is the smallest volume in which a drug may distribute, and drugs with a very small Vd (e.g., <10 L; warfarin) are mainly confined to this intravascular space. Drugs with low lipid solubility may distribute throughout total body water in the extracellular compartment and have a relatively small Vd (12 to 20 L; gentamicin), whereas more lipophilic drugs can distribute into other body fluids and tissue and have a large Vd (>50 L; duloxetine). In individuals with kidney disease, protein-binding changes may occur, specifically with acidic drugs that bind to albumin. Decreases in serum albumin concentrations (due to malnutrition or nephrotic syndrome) and changes in plasma protein binding due to conformational changes or displacement of acidic drugs from protein binding sites with the accumulation of acidic “uremic toxins” lead to an increase in unbound drug. Phenytoin is an example of a drug with altered protein binding in advanced kidney disease. Unbound free fraction ratio (unbound concentration/total concentration) increases from approximately 10% to 20% ; however, unbound (active) phenytoin plasma concentrations remain similar to concentrations in patients with normal kidney function and normal albumin levels, as unbound phenytoin clearance by the liver increases and unbound phenytoin distributes outside the vascular system. Total phenytoin concentrations are often decreased, but this does not warrant an increased phenytoin dose because the unbound concentration that determines drug exposure and response does not appreciably change. Dosing changes should be based on unbound phenytoin concentrations (unbound or free phenytoin therapeutic range, 1.0 to 2.0 mg/L) and clinical response. Available estimating equations based on total phenytoin concentration (e.g., Winter–Tozer equation) that attempt to account for decreased serum albumin level and presence of uremia have not been shown to accurately predict free phenytoin concentrations in patient settings such as critical care, inpatient medicine or neurology services, or hemodialysis. Thus, estimating equations based on total phenytoin concentration should not be used if unbound phenytoin assays are available. The acidic protein that binds basic drugs, α1-acid glycoprotein, may be increased in patients with advanced CKD, resulting in increased binding of basic drugs and a decrease in the unbound fraction. Examples of alkaline drugs that may be affected include clonidine and propranolol.
Metabolism
Nonrenal drug metabolism occurs primarily in the liver but also in the intestine and lungs. Phase I drug metabolism involves oxidation, reduction, and hydrolysis to convert drugs to metabolites that are eliminated by the kidney or excreted by the biliary system. Phase II metabolism involves conjugation reactions of the parent drug or phase I metabolite (e.g., by glucuronidation or N -acetylation). The cytochrome P450 isoenzymes (e.g., CYPs 1A2, 2C9, 2C19, 2D6, 3A4), conjugative enzymes (e.g., uridine diphosphate-glucuronosyltransferase [UGT] and N -acetyltrans-ferase [NAT]), and intestinal and hepatic transporters are responsible for the metabolic biotransformations that occur. Influx and efflux transporters, including Pgp, OATs, and multidrug resistance–associated proteins also play a key role.
Decreased clearance of CYP3A substrates observed in CKD animal models may not be due to reduced CYP3A4 activity, but to changes in transporter activity, as demonstrated in clinical studies including patients with end-stage renal disease (ESRD). Reduced non-renal clearance and/or increased oral bioavailability of over 70 drugs has been reported in patients with CKD ( Table 17.3 ). Whether the changes in metabolism or transporter activity are specifically due to CKD or to other factors has not been determined in many cases; Fig. 17.1 summarizes what is currently known about the effects of uremia on drug metabolism and transport. The potential for accumulation of active or toxic metabolites cleared by the kidney is important to consider in patients with advanced kidney disease. Examples of drugs metabolized to active and/or toxic metabolites by the liver that accumulate in patients with kidney disease include allopurinol (to oxypurinol), meperidine (to normeperidine), and morphine (to morphine-6-glucuronide and morphine-3-glucuronide).
Acyclovir | Dihydrocodeine b , c | Nortriptyline |
Aliskiren | Desmethyldiazepam | Oxprenolol b , c |
Alfuzosin | Duloxetine | Procainamide d |
Aprepitant | Encainide | Propoxyphene b |
Aztreonam | Eprosartan | Propranolol b |
Bupropion | Erythromycin b | Quinapril |
Captopril | Felbamate | Raloxifene |
Caspofungin | 5-Fluorouracil | Ranolazine |
Carvedilol | Guanadrel | Reboxetine |
Cefepime | Imipenem | Repaglinide |
Cefmenoxime | Isoniazid d | Rosuvastatin |
Cefmetazole | Ketoprofen | Roxithromycin |
Cefonicid | Ketorolac | Simvastatin |
Cefotaxime | Lanthanum | Solifenacin |
Ceftibuten | Lidocaine | Sparfloxacin |
Ceftizoxime | Lomefloxacin | Tacrolimus |
Cefsulodin | Losartan | Tadalafil |
Ceftriaxone | Lovastatin | Telithromycin |
Cibenzolin | Metoclopramide | Valsartan |
Cilastatin | Minoxidil | Vancomycin |
Cimetidine | Morphine c | Vardenafil |
Ciprofloxacin | Moxalactam | Verapamil b |
Cyclophosphamide | Nefopam | Warfarin |
Darifenacin | Nicardipine b | Zidovudine c |
Diacerein c | Nimodipine | |
Didanosine | Nitrendipine |
a Except where noted, nearly all the listed drugs undergo oxidative metabolism mediated by CYPs.
b Drugs known to exhibit an increase in oral bioavailability as well as reduced nonrenal clearance.
c Drugs that mainly undergo O -glucuronidation.

Elimination
Overall clearance or elimination of a drug is the sum of kidney and non-renal (e.g., hepatic) clearance. Kidney clearance is the net result of glomerular filtration plus active tubular secretion minus reabsorption. Insulin is a drug that undergoes metabolism in the kidney. Determinants of kidney clearance include GFR and the contribution of tubular transporters: organic cationic transporters, OATs, and Pgp. Drug transporters are significant contributors, and the FDA has proposed that important drug transporters be identified in vitro for new drugs that undergo substantial elimination by the kidney. Drugs not bound to protein may be freely filtered at the glomerulus unless restricted by molecular weight. Secretion is the primary elimination process for high-molecular-weight drugs or those that are highly protein bound. The relative contribution of filtration, secretion, and reabsorption may be estimated by calculating the Eratio for a drug [Eratio = Renal clearance/(unbound fraction × 125 mL/min)]. An Eratio <1 indicates the drug is filtered and undergoes net reabsorption; >1 indicates the drug is filtered and undergoes net secretion; 1 indicates the drug is filtered and reabsorption is equal to secretion or the drug is exclusively filtered. These primary processes may be altered in individuals with kidney disease; the alterations are more important for drugs that depend primarily on kidney versus non-renal clearance. The relative contribution of kidney clearance to overall clearance is important in determining whether dosing adjustments are warranted.
Pharmacokinetic parameters that characterize drug disposition and are helpful for drug regimen design in patients with kidney dysfunction include the half-life, or the time required for the drug’s plasma concentration to decrease by one-half, and the fraction of the drug that is eliminated by the kidney, or fe. Gabapentin is an example of a drug that is exclusively dependent on kidney function for elimination (fe = 1). If prescribing gabapentin to a patient with advanced CKD, a prolonged half-life is predictable; therefore a dose reduction or extended dosing interval would be warranted to prevent drug accumulation and adverse drug effects (e.g., dizziness, ataxia).
General Approach for Drug Regimen Design in Chronic Kidney Disease
Some general steps may help guide clinicians in determining a dosing regimen for patients with CKD. Once a drug is selected based on the indication, available resources should be used to determine if dose adjustments are warranted. This question may be answered by evaluating the drug’s pharmacokinetic parameters. This information may be included in the approved package labeling and/or in other drug references such as Lexicomp or Micromedex. Parameters of interest include the fe, half-life, and Vd. An fe >30% generally indicates that a dosing adjustment is likely warranted for a patient with advanced kidney disease, depending on the drug. More caution is warranted if the drug has a narrow therapeutic index (e.g., enoxaparin) rather than a wider index (e.g., cephalosporins). The patient’s kidney function should be assessed using available estimating equations and considering the caveats for special populations (e.g., elderly, obese). Based on the assessment of kidney function, the dose for reduced kidney function provided by the manufacturer or other available resources may be used as a reasonable starting dose. Unfortunately, dosage adjustment recommendations from various resources often conflict, which is a source of frustration for clinicians. Renal Pharmacotherapy is a textbook of compiled drug dosage adjustment information from multiple sources, including FDA-approved product labeling and alternative dosage adjustments from primary literature and compendia such as “Drug Prescribing in Renal Failure: Dosing Guidelines for Adults and Children.” Renal Pharmacotherapy provides comments when dosing information is contradictory or not supported by evidence. References are provided for the various recommendations. Micromedex and Lexicomp also provide references for many drug dosage recommendations.
For any agent prescribed, its adverse effects should also be considered. The effect of RRT (e.g., intermittent hemodialysis, peritoneal dialysis) on drug disposition must also be assessed (see the section Considerations for Drug Removal by Renal Replacement Therapies).
A dosing regimen typically includes a loading dose (LD) and a maintenance dose (MD) to “maintain” drug concentrations within a therapeutic range at steady state. An LD is administered when the goal is to achieve a target concentration sooner than four to five half-lives, which is the time required to reach steady-state conditions. This is an important consideration for patients with CKD, as the half-lives of many drugs (and thus time to steady-state) are prolonged. The LD can be calculated as LD = desired concentration (mg/L) × Vd (L/kg) × patient weight (kg) × bioavailability. The bioavailability may range from 0 to 1; it is 1 for drugs administered intravenously. Typically, the LD for a patient with kidney disease does not differ from that for a patient with normal kidney function (some exceptions are aminoglycosides, vancomycin, digoxin). The MD is the dose needed to maintain the concentration within the therapeutic window when the drug reaches steady-state conditions and is taken consistently at constant intervals. Options for dosage regimen changes for drugs eliminated by the kidney in patients with CKD include lowering the dose, prolonging the dosing interval, or both, depending on the drug. For example, for drugs dependent on concentration for therapeutic effect (e.g., aminoglycosides, quinolones), the better option is to use the same dose as for a patient with normal kidney function, but prolong the dosing interval. For drugs dependent on time above a certain concentration (e.g., cephalosporin antibiotics depend on time above the minimum inhibitory concentration for therapeutic efficacy), maintaining the same dosing frequency but lowering the dose is more appropriate.
For many drugs, information on drug disposition (the ADME) is limited for patients with advanced CKD (eGFR/CrCL <30 mL/min), and manufacturers often recommend to “avoid use” in such patients. Duloxetine is one example. It is a serotonin/norepinephrine reuptake inhibitor used for depression, anxiety, and select chronic pain disorders, and is metabolized by the liver with <1% of unchanged drug found in the urine and most eliminated as metabolites. Although elimination by the kidney is not a clinically significant elimination pathway for the active drug, there was a twofold higher maximum concentration and area under the plasma concentration versus time curve (AUC) after oral administration of this drug to 12 individuals with ESRD. This is an example of a drug predominantly cleared by non-renal mechanisms that exhibits reduced metabolism in CKD. This increase in overall drug exposure warrants cautious use in individuals with eGFR/CrCL <30 mL/min as described in the labeling. When deciding whether or how to use such agents in individuals with kidney disease, the approved prescribing information in the drug labeling must be evaluated, along with any subsequently published pharmacokinetic studies and reports regarding use and outcomes (desired and undesired). Clinical judgment should be employed when prescribing agents with limited information for patients with advanced kidney disease, particularly those who, based on assessment of kidney function, are on the borders of dosing thresholds (e.g., eGFR/CrCL 30 mL/min or 50 to 60 mL/min).
Dosing of Select Newer Agents in Chronic Kidney Disease
Direct Oral Anticoagulants
DOACs make up a class of drugs that deserves special attention due to the risk/benefit profile in individuals with low kidney function. Drugs in this class include apixaban, betrixaban, dabigatran, edoxaban, and rivaroxaban. These agents inhibit factor Xa (apixaban, betrixaban, edoxaban, rivaroxaban) or thrombin (dabigatran) and are approved for prevention and treatment of venous thromboembolism (VTE) and atrial fibrillation ( Table 17.4 ). Betrixaban is the agent most recently approved for VTE prophylaxis. Some data support the efficacy and safety of DOACs in CKD patients not treated by dialysis, although primarily from subanalysis of larger studies and metaanalyses. With regard to safety, use of DOACs resulted in a 19% decrease in risk for major bleeding compared with warfarin in individuals with atrial fibrillation and non–dialysis-dependent CKD (eGFR/CrCL 25 to 80 mL/min) based on a metaanalysis of three randomized controlled studies. This was supported by another metaanalysis of eight studies including over 10,000 individuals with CrCL 30 to 50 mL/min receiving a DOAC for atrial fibrillation or VTE compared with warfarin. Similar efficacy outcomes (stroke, systemic thromboembolism, recurrent thromboembolism, thromboembolism-related death) and safety outcomes (major bleeding, clinically relevant nonmajor bleeding) were reported. Furthermore, significantly reduced stroke and systemic embolism and reduced major bleeding compared with warfarin were reported in a metaanalysis of 13,878 individuals with CKD (CrCL <50 mL/min, CG equation) and atrial fibrillation treated with a DOAC. Although these studies are encouraging, data regarding efficacy, safety, and pharmacokinetics are lacking overall for individuals with stage 4 to 5 CKD or ESRD. Patients with CrCL <30 mL/min were excluded from clinical trials for dabigatran, rivaroxaban, and edoxaban, and those with <5 mL/min for betrixaban. Apixaban studies excluded individuals with CrCL <25 mL/min or serum creatinine above 2.5 mg/dL.
Drug | fe | Indication | CrCL (mL/min) or sCr (mg/dL) | Dose |
---|---|---|---|---|
Apixaban a (reduce dose by 50% if patient receiving dual strong CYP 3A4 and Pgp inhibitors and apixaban dose is ≥2.5 mg twice daily) | 0.27 | Nonvalvular AF | sCr <1.5 (if weight ≤60 kg AND age ≥80 y) | 5 mg twice daily (2.5 mg twice daily) |
sCr ≥1.5 and either weight ≤60 kg or age ≥80 y | 2.5 mg twice daily | |||
Hemodialysis b (if weight ≤60 kg OR age ≥80 y) | 5 mg twice daily (2.5 mg twice daily) | |||
VTE treatment | All patients | 10 mg twice daily × 7 d, then 5 mg twice daily | ||
VTE prevention | All patients | 2.5 mg twice daily after 6 mo of treatment | ||
Betrixaban | 0.05–0.07 | VTE prevention | >30 | 160 mg followed by 80 mg once daily for 35–42 d |
≥15–≤30 | 80 mg followed by 40 mg once daily for 35–42 d | |||
Dabigatran | 0.80 | Nonvalvular AF | >30 c | 150 mg twice daily |
15−30 d | 75 mg twice daily | |||
<15 | No recommendations provided | |||
VTE treatment and prevention | >30 e | 150 mg twice daily after 5–10 d of parenteral anticoagulation | ||
≤30 or on dialysis | No recommendations provided | |||
Edoxaban a | 0.50 | Nonvalvular AF | >95 | Not recommended |
>50–95 | 60 mg daily | |||
15–50 | 30 mg daily | |||
<15 | Not recommended | |||
VTE treatment | >50 (if weight ≤60 kg or on concomitant Pgp inhibitors) | 60 mg daily after 5–10 d of parenteral anticoagulation (30 mg once daily) | ||
15–50 | 30 mg daily | |||
<15 | Not recommended | |||
Rivaroxaban | 0.36 | Nonvalvular AF | >50 | 20 mg once daily |
15–50 | 15 mg once daily | |||
<15 | No recommendations provided | |||
VTE treatment | ≥30 | 15 mg twice daily × 21 d, then 20 mg once daily | ||
<30 | Avoid use |
a Patients with a CrCL <25 mL/min (for apixaban) and <30 mL/min (for edoxaban) were excluded from clinical trials.
b Based on single-dose pharmacokinetic and pharmacodynamic study performed in eight patients with end-stage renal disease.
c Reduce dose by 50% if CrCL 30 to 50 mL/min and patient is on concomitant Pgp inhibitors.
d Do not give if CrCL less than 30 mL/min and patient is on concomitant Pgp inhibitors.
e Do not give if CrCL less than 50 mL/min and patient is on concomitant Pgp inhibitors.
Pharmacokinetics and Pharmacodynamics in Chronic Kidney Disease
The fe for approved DOACs ranges from ≈6% for betrixaban to 80% for dabigatran (see Table 17.4 ); therefore there is concern for accumulation in patients with advanced CKD or ESRD, most notably with dabigatran. Dosing recommendations depend on the drug, indication, kidney function, and use of concomitant Pgp inhibitors (due to the potential for drug interactions). Dosing criteria for all DOACS are based on eCrCL, a possible cause for concern if eGFR is used for dosing in individuals with kidney disease. Apixaban, betrixaban, edoxaban, and rivaroxaban specifically state that the CG equation was used to calculate CrCL; clinical trials with betrixaban and rivaroxaban used actual body weight in the equation. These agents are not recommended below some eGFR thresholds due to a paucity of evidence (see Table 17.4 ). The exception is apixaban, which is approved at a dose of 5 mg twice daily (the dose for an individual with normal kidney function) across the eGFR spectrum unless weight is ≤60 kg or age is ≥80 years. But this dosing recommendation is based on a single-dose pharmacokinetic and pharmacodynamic study of eight patients with ESRD on hemodialysis. In this study, a 36% increase in the AUC occurred for hemodialysis patients compared with individuals with normal kidney function. Due to concern about accumulation, a subsequent study evaluated the pharmacokinetics of apixaban at steady-state in patients with ESRD with a 2.5-mg, twice-daily dose followed by the 5-mg, twice-daily dose (each administered for 8 consecutive days) in seven and five patients, respectively. The 5-mg, twice-daily dose resulted in an AUC above the 90th percentile for individuals without kidney dysfunction—which is concerning, given that this dose is recommended in the drug labeling. However, the AUC for the 2.5-mg dose was similar to the AUC for non-ESRD patients in the initial apixaban studies requiring reduced dose based on weight and/or age. Canadian labeling does not recommend use in dialysis patients. The pharmacokinetics of rivaroxaban have also been studied in patients with ESRD. A 15-mg dose administered 3 hours postdialysis resulted in a 56% increase in AUC, and decrease in drug clearance was estimated at 35% compared with individuals with normal kidney function. The authors concluded that these changes are consistent with those observed in individuals with moderate to severe kidney dysfunction and recommended a 15-mg dose for ESRD patients. The FDA label for rivaroxaban does not recommend a specific dose adjustment, but does reference this pharmacokinetic study. Unfortunately, there are no safety or efficacy data in a large ESRD population to support any specific dose in ESRD patients.
The overall lack of evidence with DOACS in patients with CKD stage 4 to 5 or ESRD warrants caution when considering their use. The risk versus benefit compared with warfarin must be considered. These agents should be discontinued and other anticoagulants used (e.g., heparin) in patients who develop acute kidney injury (AKI). Except for dabigatran, DOACs are not removed by hemodialysis and thus are not a viable reversal method for patients with bleeding complications associated with DOACS other than dabigatran. Dabigatran has a reversal agent, idarucizumab, a monoclonal antibody fragment that binds to dabigatran and its metabolites to neutralize the anticoagulant effect; however, a reversal agent’s availability does not warrant dabigatran use in patients with a CrCL <15 mL/min (for nonvalvular atrial fibrillation) or <30 mL/min (for VTE) (see Table 17.4 ). Dabigatran is contraindicated for any use in individuals with CrCL <30 mL/min based on Canadian labeling. Antidotes for DOACs other than dabigatran are currently being developed.
Agents for Type 2 Diabetes Mellitus—Sodium–Glucose Cotransporter 2 Inhibitors
Sodium–glucose cotransporter 2 (SGLT-2) inhibitors block the SGLT-2 protein involved in 90% of glucose reabsorption in the proximal renal tubule, resulting in increased renal glucose excretion and lower blood glucose levels; they also likely increase insulin sensitivity, decrease gluconeogenesis, and improve insulin release from pancreatic beta cells. By reducing glucose and sodium reabsorption in the proximal tubule, these agents decrease glomerular hyperfiltration and reduce glomerular hypertension by reducing tubuloglomerular feedback. They also lead to modest decreases in blood pressure and weight gain through these natriuretic effects and have a negligible risk for hypoglycemia when used as a monotherapy.
Pharmacokinetics and Pharmacodynamics in Chronic Kidney Disease
As a class, SGLT-2 inhibitors share similar pharmacokinetic characteristics with excellent oral bioavailability, long elimination half-life allowing once-daily administration, metabolism mainly via glucuronidation to inactive metabolites, and low renal elimination of the parent drug. Plasma AUC for the active parent drug has been shown to increase at lower levels of kidney function ( Table 17.5 ). In vitro studies with dapagliflozin have demonstrated that the kidney, in addition to the liver, is involved in the glucuronidation to inactive metabolites. The increased AUC with empagliflozin is attributed to decreased clearance by the kidney. Although increases in plasma exposure less than twofold are commonly used criteria, suggesting that no dose adjustment is required in patients with kidney disease, the higher AUC might explain the increase in adverse effects when these drugs are given to patients with eGFRs 30 to 60 mL/min/1.73 m 2 ( Table 17.6 ). Also of note, patients receiving dialysis either do not have an increase in plasma AUC versus individuals with normal kidney function (canagliflozin), or the increase is less than for those with very low eGFRs (empagliflozin) (see Table 17.5 ). Dialysis may remove uremic substances that inhibit metabolic enzymes and transporters. Despite the increase in parent drug AUC, the pharmacodynamic response to SGLT-2 inhibitors as assessed by urinary glucose excretion declines with increasing severity of kidney impairment, as the kidneys can no longer promote glucose excretion into the urine. After 7 days of treatment with 20 mg dapagliflozin, steady-state renal glucose clearance was reduced by 42%, 83%, and 84% in type 2 diabetes (T2DM) patients with CG eCrCl 51 to 80 mL/min, 30 to 50 mL/min, and <30 mL/min not receiving dialysis, respectively, leading to progressive attenuation of the glucose-lowering effect. In Japanese patients with T2DM, 24-hour urine glucose excretion increased after administration of 100-mg and 200-mg canagliflozin doses, but in patients with eGFR 31 to 49 mL/min/1.73 m 2 , the increase was only about 70% of that in patients with normal kidney function or with mildly decreased eGFR (≥ 80 mL/min/1.73m 2 ).
Drug | Bioavailability (%) | Volume of Distribution (L) | ∗Plasma Protein Binding (%) | Metabolism | Renal Excretion | Comments |
---|---|---|---|---|---|---|
SGLT-2 Inhibitors | ||||||
Canagliflozin | 65 | 84 | 99 | Hepatic and renal: extensive via O-glucuronidation to two inactive metabolites | 33% (<1% as parent drug) | Single dose canagliflozin 200 mg increased parent drug AUC 15% (eGFR 60–<90); 29% (eGFR 30–<60); 53% (eGFR 15–<30) but no AUC increase in HD vs. normal kidney function. |
Dapagliflozin | 78 | 118 | 91 | Hepatic and renal: extensive via O-glucuronidation to 1 inactive metabolite. In vitro data show that dapagliflozin 3-O-glucuronide is formed in both the kidney and liver. | 75% (<2% as parent drug) | Dapagliflozin 20 mg for 7 d increased parent drug AUC 32% (CrCl 51–80); 60% (CrCl 30–50); 87% (CrCl <30) vs. normal kidney function. Not studied in dialysis. |
Empagliflozin | 78 | 74 | 85% normal kidney function, 81% CKD | Limited via hepatic glucuronidation to three minor metabolites with unknown activity (<10% each metabolite); 41% eliminated as parent drug in feces | 54% (11%–19% as parent drug | Single dose empagliflozin 50 mg increased parent drug AUC 18% (eGFR 60–<90), 20% (eGFR 30–<60), 66% (eGFR <30), 48% (dialysis). |
DPP-4 Inhibitors | ||||||
Alogliptin | ≈100 | 417 | 20 | Hepatic: limited metabolism, via CYP2D6 and CYP3A4 to active metabolite (<1%) | 76%, (60%–71% parent drug) | |
Linagliptin | 30 | 1110 | 70–99 | Hepatobiliary: limited to an inactive metabolite | <7% parent drug | Linagliptin is the only DPP-4 inhibitor not primarily excreted by the kidneys but via hepatobiliary excretion. A PK study of linagliptin and post hoc analyses of trough plasma levels with linagliptin 5 mg daily for 24–52 wk showed that decreased GFR has negligible effects on total drug exposure. |
Saxagliptin | 75 | 198 | Negligible | Hepatic: primarily via CYP450 3A4/5 enzyme subsystems to an active metabolite. | 60%, 24% parent drug; 36% as active metabolite | Saxagliptin is also metabolized to an active metabolite, 5-hydroxy-saxagliptin, which exerts significant DPP-4 inhibition (half as potent as the parent compound), and both are excreted primarily by the kidneys. |
Sitagliptin | 87 | 198 | 38 | Hepatic: minimal, primarily CYP3A4 with contribution from CYP2C8. | 87%, 79% parent drug | |
GLP-1 Receptor Agonists | ||||||
Albiglutide | ND | 11 | ND | Protein catabolism degradation to amino acids. | ND | Once-weekly albiglutide assessed in a pooled analysis of four phase III, randomized, active, or placebo-controlled, multiple-dose studies of patients with CKD (eGFR ≥15–<90) showed modest increases in albiglutide plasma concentrations and a trend for a more potent glucose-lowering effect as eGFR decreased. |
Dulaglutide | 47 (1.5-mg dose)–65 (0.75-mg dose) | 17.4–19.2 | ND | Protein catabolism degradation to amino acids. | ND | |
Exenatide | ND in humans 65–76 (animal data) | 28.3 | ND | Protein catabolism degradation to amino acids. | Predominantly renal: glomerular filtration with subsequent proteolytic degradation | Baseline CrCL was determined to be the most significant predictor of steady-state concentration of exenatide once-weekly formulation. |
Liraglutide | 55 | 13 | >98 | Protein catabolism degradation to amino acids. | 6%, 0% parent, 6% degraded | Liraglutide half-life was not increased and clearance was not decreased in 30 subjects with varying stages of CKD administered a single dose of 0.75 mcg SQ supporting observation that the kidneys are not a major site for elimination or degradation. |
Lixisenatide | ND in humans | 100 | Protein catabolism degradation to amino acids. | Predominantly renal: glomerular filtration with subsequent proteolytic degradation | Limited PK data in patients with CKD are available. |
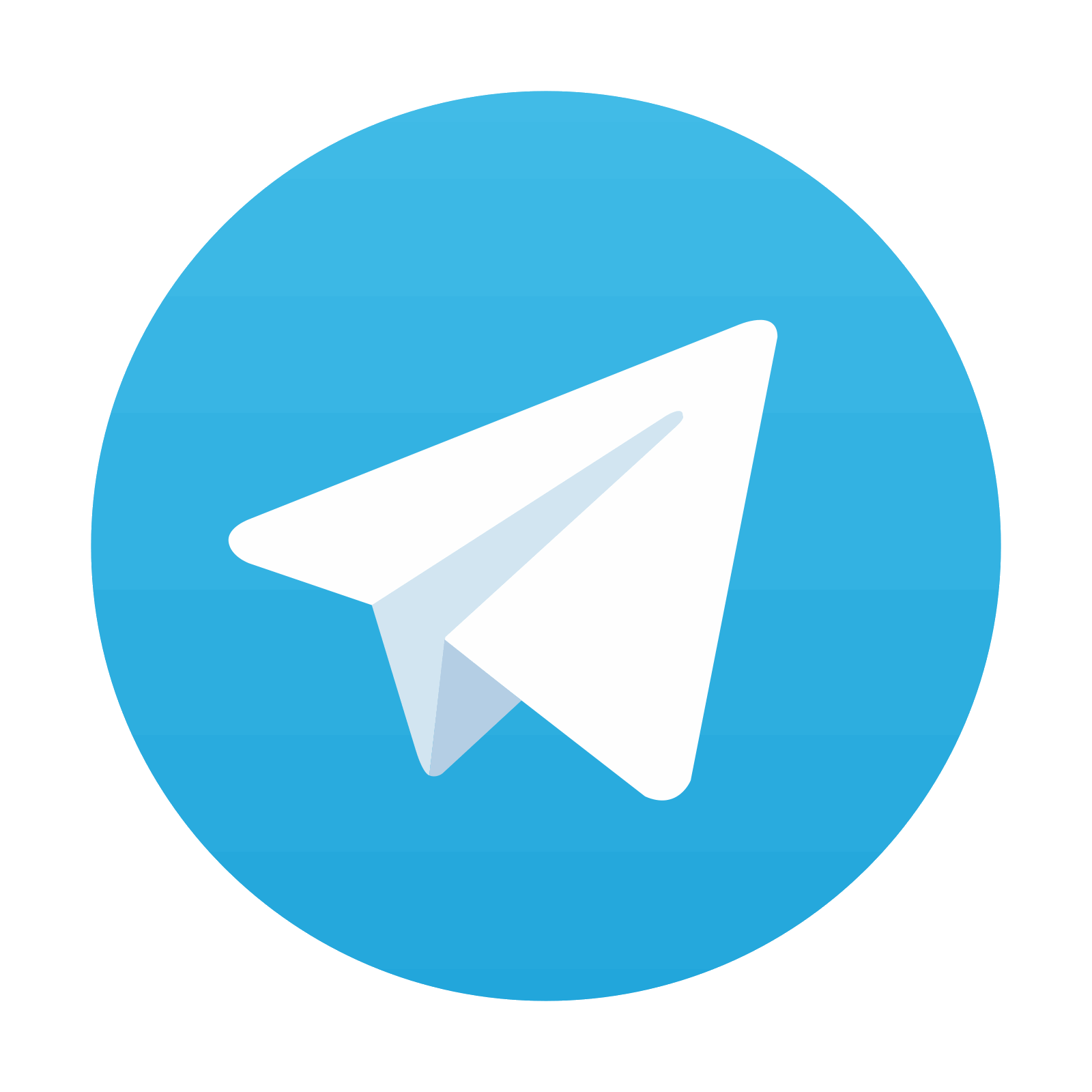
Stay updated, free articles. Join our Telegram channel
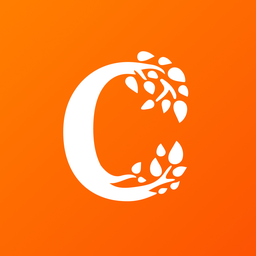
Full access? Get Clinical Tree
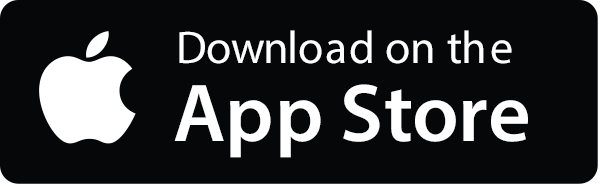
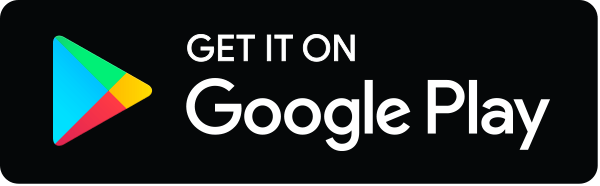
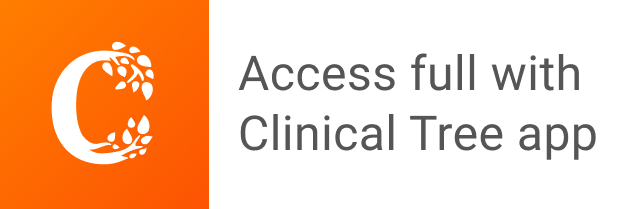