Abstract
The mechanisms that orchestrate the development of a multicellular organism from a single common ancestor cell have fascinated people for centuries. We are still a long way from truly understanding the robust programs that allow building of our specialized organs that regulate digestion, respiration, and hemodynamics and that guard us from invasion by other unsolicited organisms. However, in the past three decades, many of the genes that play a role in patterning our bodies have been identified. We have learned much about the mechanisms involved in development by characterizing the nature of these patterning genes and the way they work together. In this chapter, we will discuss patterning mechanisms, introduce the Hedgehog pathway and then summarize our current understanding of the role of Hedgehog signaling in development and maintenance of the gastrointestinal (GI) tract.
Keywords
Hedgehog, Morphogens, Development, Homeostasis, Cancer
Abbreviations
BMP
bone morphogenetic protein
Disp
dispatched
d.p.c.
days post coitum
Fgf
fibroblast growth factor
Fu
fused
Hh
Hedgehog
Hhip
Hedgehog interacting protein
HSC
hepatic stellate cell
IFT
intraflagellar transport
PanIN
pancreatic intraepithelial neoplasia
PDAC
pancreatic ductal adenocarcinomas
Ptch
patched
Smo
smoothened
Sufu
suppressor of fused
Tgfβ
transforming growth factor β
4.1
Patterning
We are built from hundreds of specialized cell types that make up our organs. The development and postnatal maintenance of these cell lineages are tightly controlled and incredibly stable in time. Cells are capable to go through major shifts in protein expression in time in response to physiological and environmental cues without changing cellular phenotype. Each cell type is defined by the expression of a unique combination of proteins transcribed and posttranscriptionally modified from the templates of our 20,000–25,000 genes. One of the central questions in biology is how the information contained in these genes can translate into a spatially complex multicellular organism. The idea that such complexity is possible because cellular phenotype is coupled to cellular position is at least 100 years old. We have learned much about the proteins involved in the specification of cellular position from developmental biologists that have used models such as the regulation of segmentation of the Drosophila larval epidermis, development of the vertebrate limb bud, neurogenesis, and somite formation.
Most of the evolutionary conserved signaling pathways that act to couple cellular position to cellular phenotype have been first identified in genetic screens in Drosophila most notably the systematic screens performed by Nusslein-Volhard and Wieschaus. The type of molecules that have emerged from these genetic screens can be classified in two broad categories, intrinsic and extrinsic factors. Intrinsic signals such as kinases, phosphatases, and transcription factors function within the cell and thus act in a cell-autonomous manner. Extrinsic signals are soluble or transmembrane factors that act among cells and regulate cell-nonautonomous processes. Extrinsic information is critical for a complex tissue composed of multiple different cell types to organize appropriately. These tissues contain gradients of soluble factors that act in a concentration-dependent manner to control cell fate. Such factors have been termed morphogens. This concept was most clearly voiced by Wolpert in the 1960s, who developed the French Flag model ( Fig. 4.1 ). A morphogen receiving cell has one or more concentration thresholds that result in the expression of a distinct set of target genes. Thus, morphogen concentration gradients confer positional information by specifying distinct cellular phenotypes in a field of receiving cells depending on their distance from the source of the signal. Signaling molecules from the hedgehog (Hh)-pathway, the Wnt-pathway, the transforming growth factor (TGF) β-superfamily, and receptor tyrosine kinase pathways have been shown to act as morphogens in several models of development and are used in the regulation of cell fate throughout evolution and in most developing tissues. This chapter will summarize our current understanding of the Hh-pathway and its role in the development and postnatal maintenance of the GI tract.

4.2
The Hedgehog-Pathway
4.2.1
Hedgehog (Hh) Genes
The Hh gene was identified in the first systematic screen for patterning genes in Drosophila . In the larvae of wild-type Drosophila , a band of bristles called denticles is present across the anterior half of each segment, whereas the posterior half is smooth (the so-called naked cuticle). In screening for mutations that affect the segmental pattern of the Drosophila larva, Nüsslein-Volhard and Wieschaus identified a group of mutants that affected the patterning within the segments but at the same time left the number of segments unaltered. In one of these segment polarity mutants, the posterior half of each segment failed to develop, resulting in a larva that is entirely covered by denticles ( Fig. 4.2 ). This phenotype gave the larva the aspect of a Hedgehog ( Hemiechinus ), hence the name. After much effort, the nucleotide sequence of the Hh gene was finally obtained, and the mammalian Hh homologues were identified. Echelard et al. identified three Hh genes in the mouse. They were named Sonic Hedgehog ( Shh ), after a popular Sega computer game character, Desert Hedgehog ( Dhh ), after an Egyptian species of Hedgehog ( Hemiechinus auritus ), and Indian Hedgehog ( Ihh ), a Hedgehog species endemic in Pakistan ( Hemiechinus micropus ). Riddle et al. found that Shh protein mediates the action of the zone of polarizing activity (ZPA) in the chick limb bud ( Fig. 4.3 ). The ZPA is a region at the posterior margin of the chick limb bud responsible for normal anteroposterior patterning. Shh was shown to colocalize with this previously described region and to alter limb patterning in a way similar to ZPA grafting. At the same time, a Hh homologue was identified in zebra fish ( Danio rerio ) and shown to be expressed in tissues with organizing activity. In 1995, a new Hh homolog was found in zebra fish and was named Tiggy-Winkle Hh, after a character in a children’s book. Most phyla of the eukaryotic kingdom have now been shown to contain Hh homologues, and the expression of vertebrate Hh genes has been detected in a variety of tissues during development. Shh , Dhh , and Ihh are highly conserved between mouse and humans. Unfortunately, little is known about factors that control the transcription of Hh genes, particularly in the gut.


4.2.2
The Unusual Posttranscriptional Fate of Hh Proteins
In order for the Hh protein to act as a potent morphogen, it needs to be processed in different ways ( Fig. 4.4 A). After removal of the signal sequence, approximately 45 kDa large Hh proteins are cleaved auto-catalytically yielding an 19 kDa N-terminal fragment that contains all the signaling functions, and a 26 kDa C-terminal fragment that has no other function than to catalyze the cleavage. The unusual posttranslational processing of Hh proteins is not limited to this autocatalytic processing of the primary translated product. In the course of the cleavage, the Shh protein is covalently palmitoylated. Palmitoylation in the secretory pathway is atypical as palmitoylation is usually restricted to the cytoplasm and thus only affects proteins without a signal peptide and not proteins entering the secretory vesicular pathway. Palmitoylation is essential to both activity of the Hh protein and the signaling range. Palmitoylated recombinant Shh has an approximately 30-fold higher biological activity than unpalmitoylatable mutants suggesting that this acylation is important for enhancing biological activity. Even more atypical than the palmitoylation is the covalent attachment of a cholesterol moiety to the C-terminal part of Hh-N, making Hh proteins the only established example of protein sterolation. Conflicting reports have been published on the role of the cholesterol moiety in Hh signaling. The lipid-modified (processed) Hh signaling domain (Hh-Np) is hydrophobic and thus able to bind to the cell membrane where it mediates local signals. Dispatched (Disp), a 12-pass transmembrane protein with a sterol-sensing domain, is required for the release of Hh-Np from the cell membrane. Disp −/− mutant mice present with a similar phenotype as mice that lack the signaling receptor Smoothened (Smo), suggesting Disp is required for all Hh signaling.

Despite the fact that lipid-modified Hh is not soluble, it is known to form a concentration gradient through a tissue and is able to activate long-range target gene expression over at least 8–10 cell diameters. Different models have been proposed to explain how the lipophilic Hh can spread through an aqueous tissue ( Fig. 4.4 B). Several laboratories have shown that both cholesterol and palmitate modifications are required to mediate multimerization complexes of NH 2 -terminal Hh proteins. The lipid moieties are thought to be embedded in the core of these complexes, in analogy to micelles. A second model proposes that the lipid moieties of Hh-Np associate with lipoprotein particles. The role of lipoproteins as vehicles for long-range Hh targets has been demonstrated by several groups who showed that depletion of circulating lipophorin particles, the Drosophila equivalent of lipoproteins, reduces the range of Hh signaling. Distribution of the lipid-modified Hh protein is further facilitated by interactions with proteoglycans, heparin, and chondroitin sulfates. Besides being the receptor for the Hh ligand, Ptch has been shown to function as a lipophorin receptor. Furthermore, Callejo et al. demonstrated that Ptch modulates intracellular lipid homeostasis, independently of its role in the regulation of Hh signaling. A link between Hh signaling and lipid metabolism may have important implications for vertebrate Hh signaling in diseases as atherosclerosis and adipogenesis-related diseases, such as osteoporosis, lipodystrophy, diabetes, and obesity.
4.2.3
The Receptor Complex
Hh signal transduction is highly unusual, containing many features unique to this signaling system. The Hh-binding receptor Patched (Ptch) is a 12-transmembrane protein with two large hydrophilic extracellular loops that mediate Hh binding. However, Ptch does not convey the Hh signal to the intracellular components of the pathway itself like a conventional receptor. Rather, binding of Hh to Ptch alleviates the inhibitory effect of Ptch on another membrane receptor, the 7-transmembrane protein Smoothened (Smo). Two Ptch genes exist in vertebrates, Ptch1 and Ptch2 , but Ptch1 mediates most, if not all, effects of Hh signaling. In the absence of Hh, Ptch inhibits signaling by Smo. Upon binding of Hh to Ptch, this inhibition is alleviated and Smo will activate Hh target gene transcription ( Fig. 4.5 ). Ptch1 is a transcriptional target of Hh signaling and acts in a negative- feedback loop to restrict the range of Hh signaling in a tissue. In addition to Ptch, several other proteins have been identified that bind Hh and modulate signaling. Hh-interacting protein ( Hhip ) is a transcriptional target that acts as a negative regulator of Hh signaling by binding Hh on receiving cells. An additional class of Hh-binding proteins has been identified in Drosophila , interference Hedgehog (Ihog), and Brother of IHog (Boi), which interact directly with Ptch and have been shown to be essential for Hh transduction and target gene expression. Two vertebrate homologues are known, Cdo and Boc. These Hh-binding proteins facilitate binding and positively regulate signaling.

The mechanism of the inhibitory action of Ptch on Smo is only partially understood. Ptch belongs to the resistance-nodulation-division (RND) family of small molecule pumps and is closely related to the Niemann-Pick C1 (NPC1) protein, a transport protein involved in cholesterol homeostasis, which can act as a proton driven pump to flux small lipophilic molecules across the bilayer. A plausible hypothesis is that Ptch functions as a pump that regulates the availability of a compound that mediates the function of Smo. Mammalian Smo activity can be modulated by binding to lipophilic molecules structurally related to sterols, and it is thought that a structurally related endogenous ligand for Smo might exist. There is increasing evidence indicating that sterols may act as endogenous activators of Smo. Sterols are required for Hh signaling because sterol depletion or genetic defects in cholesterol biosynthesis results in inadequate signaling. Furthermore, some oxysterols, generated by cholesterol oxidation, are able to activate Smo by binding to the extracellular, cysteine-rich domain (CRD) of Smo. Recently, cholesterol itself has been proposed as an endogenous molecule to bind and activate Smo by causing a conformational change of the CRD. However, if cholesterol is also a substrate for the predicted transporter activity of Ptch1 at the side of the primary cilium is still a matter of active investigation. For a complete review of the Hh signaling molecules see Ref. .
4.2.4
Signal Transduction and Target Genes
The regulation of Smo activation and downstream Hh signaling have been only partly revealed. Most studies were performed in Drosophila ; however, this part of the pathway seems to have diverged considerably during evolution from Drosophila to mammals. For example, Drosophila do not have cilia, whereas in vertebrates, the Hh-pathway is dependent on the primary cilium. In Drosophila , fused (Fu) is essential for Hh signaling, whereas Fu is dispensable for Hh signaling in mammals. Therefore, we will focus on experiments performed in vertebrates where possible. In Drosophila , the Hh-pathway acts through a single transcription factor cubitus interruptus (Ci). In vertebrates, three Ci homologues have been identified termed Gli-1 to Gli-3. Gli2 acts mainly as a transcriptional activator, whereas Gli3 can also be processed into a transcriptional repressor. Gli1 is a transcription factor as well as a target of Hh signaling and functions as an amplifier of activated Hh signaling. Primary cilia have been shown to play a major role in Hh signaling in vertebrate systems. They are specialized subcellular signaling centers that are required for the response to developmental signals ( Fig. 4.6 ). Evidence that Hh signaling depends on cilia first came from a phenotype-based mutation screen, in which genes encoding components of the intraflagellar transport (IFT) machinery were disrupted. IFT proteins are responsible for maintenance of the cilia and for transport of particles along the axoneme, a microtubule-based cytoskeletal structure in the inner core of the cilium. Mutations in IFT proteins have been shown to lead to similar phenotypes as mutations in the Hh signaling pathway. IFT proteins act downstream of membrane proteins Ptch and Smo and upstream of the Gli transcription factors, for a review see Ref. . The importance of cilia in vertebrate Hh signaling was further demonstrated by the fact that all key components of the Hh-pathway are enriched in cilia. Both Ptch1 and Smo show dynamic, Hh-dependent trafficking in cilia. In the absence of Hh ligand, Ptch1 is localized at the cell surface of the ciliary base where it waits to bind extracellular Hh ligands. Although Smo circulates in and out of the cilium, Smo is mainly localized outside of the cilium at the cell membrane and intracellular vesicles. Upon binding of Hh to Ptch1, the receptor-ligand complex is internalized in endosomal vesicles, Smo accumulates at the ciliary membrane, and Hh target genes are activated by Gli proteins.

Gli2 and Gli3 are the major Glis to transduce the Hh signal in the gut. Gli2, which functions predominantly as activator of Hh signaling, shifts from a primarily cytoplasmic localization to the distal cilium tip and the nucleus, where it activates gene expression. In the absence of Hh, Gli3 is restrained to the cytoplasm by Suppressor of fused (Sufu) and proteolyzed into a truncated transcriptional repressor. Gli2 can also function as a repressor of Hh target expression; however, processing of Gli3 is much more efficient than that of Gli2. Sufu-Gli3 interaction is therefore a major control point in the Hh-pathway. Genetic inactivation of Sufu leads to constitutive activation of Hh signaling and in humans Sufu is known as a tumor suppressor gene.
Gli3 is converted into a transcriptional repressor by a complex of protein kinases. PKA initiates a partial proteolytic processing reaction in which PKA and subsequently CK1 and GSK3β phosphorylate Gli3. This phosphorylation can result in ubiquitination and partial degradation that generates a truncated version of Gli3 that acts as a dominant negative Gli. In the presence of Hh, Gli3, and Sufu dissociate, and an active form of Gli3 enters the nucleus to activate target gene expression. It is unknown by which mechanism the Hh signal counteracts Sufu’s repression of Gli activity, but some studies suggest that Smo activation can reduce PKA activity by activation of G protein Gα1 functions, leading to dephosphorylation and degradation of Sufu and subsequent dissociation of the Gli3-Sufu complex. Another protein that plays a role as a regulator of Hh signaling is Kif7, the homolog of Drosophila Cos2. Activity of Kif7 is dependent on the presence of the primary cilium. Upon Hh signal activity it traffics along the ciliary axoneme and localizes to the distal tip of the cilium where it controls cilium architecture creating an optimal environment for Sufu and Gli3 to interact. Kif7 behaves similarly to Drosophila Cos2 as a predominantly negative regulator of Hh target genes and probably serves as a scaffold for the production of Gli repressors. For a comprehensive review on the Hh signaling pathway see Ref. .
Following the release of transcriptional repression, Hh target genes will be transcribed. Regulation of target genes differs in time and between organs. Ptch1, Gli1, and Hhip are the most widely used as readouts for Hh-pathway activity, but many more Hh targets have been described. Ptch1 and Hhip are not only targets of Hh signaling but also part of a negative feedback loop and diminish the Hh response. On the other hand, Gli1 functions as transcriptional target that further enhances signaling and thus is part of a positive feedback loop.
4.3
The Role of Hh Signaling in the Developing Gut
4.3.1
Development of the GI Tube
The GI tract develops from a simple tubular structure composed of cells derived from all three germ layers. Cells from the endoderm will form the epithelial layer; mesodermal cells differentiate into mesenchymal cell types such as myocytes and fibroblasts whereas neural cells originate from the ectoderm and innervate the GI tract. The gut tube acquires regional specialization along the longitudinal axis of the developing embryo. The fate of cells that form this tube is restricted to become part of one of the roughly four specialized tissue units of the esophagus, stomach, small intestine, and colon. Other small groups of cells bud from the original tube to form the thyroid, thymus, lungs, liver, and pancreas. A second axis of specialization is the cross sectional or vertical axis. The constant renewal of epithelial cells in the adult occurs along this vertical axis. Most patterning mechanisms along these axes involve cross talk of morphogens between cells of the different germ layers.
Hh signaling plays a role in the specification of cellular fate along both the longitudinal and the vertical axis and its regulation is involved in budding of gut tube-derived organs. The endodermal cell layer expresses Hh family members Shh and Ihh. We will discuss our current understanding of the role of Hh signaling in GI development in three sections that will cover the three segments of the gut and its derivatives: foregut (esophagus, trachea, lungs, thymus, stomach, liver, and pancreas), the midgut (small intestine), and hindgut (colon and anus). For a detailed description of gastrulation, which takes place early in embryological development, and axis formation see Ref. .
4.3.2
Hh Signaling and Foregut Development
4.3.2.1
Development of the Esophagus and Trachea
After formation of the foregut primordium, a central trachea bud and two adjacent ventrolateral lungbuds form from the ventral wall of the foregut at E9.5. In the mouse foregut, Shh mRNA expression is initiated at 9.5 days post coitum (d.p.c.) in the endoderm of the foregut primordium and will extend along the ventral wall of the developing foregut toward the midgut. Expression of Shh in the murine esophagus has been described until at least 15.5 days post coitum (d.p.c.). Signaling is from endoderm to mesoderm, as Gli transcription factors are exclusively expressed in the foregut mesoderm. At some later point in development, expression of Shh is downregulated in the esophagus as no Shh expression is found at 18.5 d.p.c. In the elongating trachea, Shh expression is initially lost at 11.5 d.p.c. but then reappears around 15.5 d.p.c. In contrast, no Shh is expressed by the cells of the thyroid, which forms as a primordium at 9 d.p.c., is completely dissociated from the gut tube by 11.5 d.p.c. and descends to its final position until 15 d.p.c. Ihh is not expressed at any point in foregut development, and this is confirmed by the fact that Shh and Gli mutant mice show similar foregut phenotypes (see below).
The role of Shh signaling in foregut development is revealed in Hh-pathway mutant mice ( Table 4.1 ). In Shh −/− mice, the formation of the tracheal bud is delayed and the lungs become hypoplastic. The normal elongation of the trachea fits well with the fact that no Shh expression is found during this phase. The hypoplastic lungs suggest a role for Shh signaling in branching morphogenesis. The esophagus fails to develop normally, only a small esophageal remnant is found in Shh −/− mice at later stages of development. Since Shh has been shown to signal mainly to the mesenchyme during development and seems to act as a growth factor for mesenchymal cells, it may be that the esophagus degenerates because of a lack of mesenchymal cell growth to support its epithelium. As a result, the stomach seems to connect to the respiratory tract in Shh null mice.
Esophagus | Stomach | Intestine | Anorectum | Reference | |
---|---|---|---|---|---|
Shh −/− | Esophagus and trachea fail to separate, tracheoesophageal fistula | Anterior expansion of glandular stomach, increased gland fission | Gut malrotation, duodenal villus overgrowth, abnormal neuronal differentiation | Imperforate anus, fused anorectum-urinary tract | |
Ihh −/− | No gross abnormalities | No gross abnormalities | Gut malrotation, hypoplastic villi, segmental absence of neurons, Hirschsprung’s-like colonic phenotype | No gross abnormalities | |
Gli1 −/− | Grossly normal | Grossly normal | Grossly normal | Grossly normal | |
Gli2 −/− | Hypoplastic trachea and esophagus, hypoplastic lungs, lobulation defect right lung | No gross abnormalities | ND | Imperforate anus, fectovesical fistula | |
Gli3 −/− | ND | Anterior expansion of glandular stomach, increased glandular growth | ND | Anal stenosis | |
Gli2 −/− Gli3 +/− | Esophagus and trachea fail to separate, tracheoesophageal fistula | ND | ND | Persistent cloaca, common outlet gut-urinary tract | |
Gli2 −/− Gli3 −/− | Complete atresia of esophagus | ND | ND | Persistent cloaca, common outlet gut-urinary tract | |
HKcre-Shh fl/fl | Foveolar hyperplasia, hypergastrinemia, disrupted differentiation, hyperproliferation of the surface pit epithelium | ||||
HKcre-sHhip | Hypochlorhydria, repression of Shh gene expression | ||||
Villin-Hhip | Hypoplastic branching villi, precursor cell accumulation, crypts on villi | ||||
VillinCre-Ihh fl/fl | Crypt fissioning, villus branching, ectopic crypt formation, disruption of mesenchymal compartment, development of inflammation |
The importance of Hh signaling in foregut development was confirmed in Gli mutant mice. Although Gli3 −/− mice have a normal trachea and esophagus, in Gli2 −/− mutant mouse, the esophagus has a very small lumen, few mesenchymal cells, and fails to develop a small muscle layer. The lungs in the Gli2 −/− mutant mouse are hypoplastic and have only one right lobe instead of the four lobes that are normally found. Although the trachea elongates normally in the Gli-2 mutant mouse it does appear hypoplastic at 18.5 d.p.c. suggesting that the induction of Shh expression in the trachea at 15.5 d.p.c. is involved in its growth along the vertical axis. The Gli2 −/− Gli3 +/− mouse has a phenotype that is more severe than the Gli2 −/− mouse and seems identical to the Shh null mutant. Therefore, although Gli3 is not necessary for normal foregut development, it can rescue part of the phenotype in Gli2 −/− mice. Interestingly, the Gli2 −/− Gli3 −/− phenotype is more severe than the Shh null phenotype demonstrating no evidence of lung, trachea, or esophagus development at all. This suggests that Gli signaling in foregut development is not entirely Shh dependent. Some effectors of Hh signaling have been found by the similarity of their null phenotype to the Hh-pathway mutant phenotypes. The forkhead transcription factor Foxf1 seems to play a role as a mesenchymal effector downstream of Gli signaling. Foxf1 is expressed in the splanchnic mesoderm. Foxf1 +/− mutant mice display a phenotype comparable to Shh mutant mice with esophageal atresia and tracheoesophageal (TE) fistula. It was further shown that ectopic Shh expression activates Foxf1 expression whereas Foxf1 expression is lost in Shh −/− mice.
In conclusion, Hh signaling from the endoderm to the mesoderm seems to play an essential role in the normal separation of trachea and esophagus, maintenance and growth of the esophagus, and growth of the lungs. It seems to play this role through regulation of mesenchymal growth through induction of Foxf1.
4.3.2.2
Development of the Thyroid
Although the thyroid primordium is correctly specified at 9 d.p.c. in the absence of Hh signaling, careful examination of the thyroid phenotype in the Shh null mouse does reveal a role for Shh signaling at later stages of thyroid development. Thyroid morphogenesis is initiated at E8.5 when the thyroid primordium buds of the ventral wall of the pharyngeal foregut endoderm that lies in close contact with the aortic sac endothelium. The thyroid primordium buds of the foregut endoderm and becomes completely separated at 11.5 d.p.c. At E13.5, the thyroid is at its final position after which it separates into two lobes that develop in close contact with the carotid arteries. At E15.5, the thyroid organizes into a follicular structure and genes involved in thyroid hormone production are induced. In Shh −/− mice, the thyroid primordium forms but separation from the foregut is delayed, as it remains connected through a narrow stalk initially. The thyroid does not separate into two distinct lobes but becomes a single unilateral mass, the size of a single thyroid lobe in control mice. The single thyroid gland of Shh null mice formed normal follicles that contained apparently normally differentiating thyrocytes. The fact that no Shh or Ptch expression was detected in the thyroid at any point of development suggests that the thyroid phenotype in Shh −/− mice is indirect. This was shown by Alt et al. who demonstrated that the phenotype is a result of defective patterning of the cervical vasculature. Interestingly, small foci of ectopic thyroid tissue were found in the tracheal tube at 15.5–17.5 d.p.c. in the Shh −/− mouse, suggesting that the induction of Shh in the trachea not only plays a role in tracheal growth along the vertical axis (see above) but also acts to suppress thyroid cell fate in the trachea.
4.3.2.3
Development of the Stomach
During development of the stomach, the gastric epithelium is patterned into three distinct zones along the proximal-distal axis and in precursor cell and differentiated cell compartments along the vertical axis. In humans, the whole stomach consists of columnar glandular epithelium; in mice, the proximal one-third is lined with squamous epithelium and is known as the forestomach. The proximal part of the stomach is the fundic or zymogenic zone, the distal part is the antral zone. The glandular epithelium forms tubular structures that invaginate into the underlying mesoderm. These tubules contain two cell populations: the superficial cells, which form the pit or foveolus, are similar throughout the stomach and produce soluble mucins that contain a protein backbone encoded by the MUC5AC gene. The cells that form the bottom of the tubules form the glandular region, the gland proper, and have both exocrine and endocrine functions. In the adult, both cell populations originate from a common precursor cell compartment, the isthmus, in between the pit and the gland region from where daughter cells migrate in two directions ( Fig. 4.7 ). The gastric glands of the proximal (fundus) and distal (antrum) stomach are functionally distinct. From E11.5 until after the onset of gastric epithelial differentiation at E15.5 Shh and Ihh are expressed in opposing gradients in the mouse. Shh expression is high in the forestomach and weaker but detectable in the distal stomach, whereas Ihh is expressed in the distal stomach that will give rise to corpus and antrum and is undetectable in the forestomach. No gross abnormalities are reported in the stomachs of Ihh −/− mice ( Table 4.1 ) suggesting Ihh protein does not play a major role at this time point. Ptch1 , Gli1, and Gli2 are all exclusively mesenchymal at E14.5. At E18.5, high expression of both Shh and Ihh is found in glandular epithelium of the stomach and at birth Ptch1 , Gli1 , and Gli2 are robustly expressed in mesenchymal cells, with prominent activity at the antropyloric border. Fgfr2b −/− and Fgf10 −/− mice lack expression of Ihh in the stomach that shows that Ihh expression depends on Fgf signaling.

Expression of Ptch1 is in the mesoderm and mirrors that of Shh. As most of the major events in cytodifferentiation occur postnatally in the mouse stomach, the use of Hh-pathway mutant mice in studying gastric development is hampered by the fact that these mice die well before birth. Shh signaling seems to specify the forestomach domain as Shh −/− mice have a reduction of squamous epithelium that forms the forestomach, whereas Activin mutant, Gata4 −/− , Fgfr2b −/− , and Fgf10 −/− mice in which Shh expression is expanded from the anterior to the posterior stomach present with a concomitant expansion of the forestomach. Fgf10 is expressed in the mesoderm of the glandular stomach and signals to the endoderm where Fgfr2b and Gata4 are expressed. Experiments with different knockout mice demonstrated that Fgf10 signaling is upstream of Gata4 in the restriction of Shh expression to the forestomach. Possibly, there is a role for Activin downstream of Fgf signaling and upstream of Gata4. Shh also plays a role in the glandular stomach as in Shh −/− mice (but not in Ihh −/− mice) the epithelium of the glandular stomach is hyperplastic ( Table 4.1 ). It has been reported that intestinal transformation occurs in these mice, but this was denied by another group who found normal expression of gastric markers, no goblet cells, no brush border and no expression of intestinal enterocytes markers.
In conclusion, the available evidence suggests that high levels of Shh expression in the forestomach inhibit gland formation, resulting in squamous epithelium that lacks glands. Much lower levels of Shh in the glandular stomach, due to inhibition of Shh by Fgf10, allow gland formation, but restricts gland branching. Due to early lethality of knockout mice and relatively late completion of the cellular differentiation and glandular growth in the developing stomach inducible and conditional inactivation of Hh-pathway components would be valuable in further analysis of Hh signaling in gastric development.
4.3.2.4
Development of the Pancreas
The pancreas is an endodermal derived gland with both endocrine and exocrine functions. The bulk of pancreatic tissue (approximately 95%–99%) is composed of exocrine acinar cells that produce digestive enzymes and pancreatic ducts that are lined by duct cells. The rest of the pancreas consists of small clusters of endocrine cells or Islets of Langerhans. Each cluster of endocrine cells has a core of insulin producing β-cells surrounded by glucagon producing α-cells, somatostatin producing δ-cells, and pancreatic-polypeptide cells. The pancreas develops from two distinct primordia that originate at the ventral and dorsal side of the gut tube, which develop between E8.5 and E9.5. At this time, the first endocrine cells are also detectable.
At 10.5 d.p.c., Shh and Ihh are expressed uniformly throughout the endoderm of the gut tube with the exception of pancreatic precursor cells that are distinguished by their Pdx-1 positivity. When a Pdx-1 promoter was used to drive ectopic Shh expression in the pancreatic epithelium, development of the pancreatic endoderm and mesoderm was disturbed. The mesenchyme contained α-actin positive smooth muscle cells and c-kit positive cells of Cajal. Both cell types are normally found in intestinal mesenchyme. The pancreatic buds in transgenic animals did contain endocrine and acinar cells, but these failed to cluster into islets and acinar structures and produced mucins that are typical for intestinal epithelial cells. More recently, activating mesenchymal Hh signaling by deletion of Ptch1 from all mesenchymal cells resulted in disrupted growth of the pancreatic epithelium, influencing both the endocrine and exocrine compartment. Furthermore, islet morphology was changed, whereas mesenchymal growth was increased. These data suggest that during early pancreatic development suppression of Shh signaling is especially important for normal development of the pancreatic epithelium and mesoderm. Data from Xenopus further support this concept. Xenopus injected with a constitutive active form of the Hh receptor Smo completely lack a pancreas, a phenotype more severe than that of the Pdx-1 -Shh mouse. This may be due the fact that their transgenic mouse expressed Shh behind a pancreas specific promoter and that the ectopic Shh transcription therefore starts after the initial specification of a pancreatic domain has already been completed. Remarkably, Cervantes et al. demonstrated that upregulation of the Hh-pathway by ectopically overexpressing Gli2 using the Pdx-1 promoter could only be achieved when the primary cilia were concomitantly ablated, suggesting a critical role for these organelles in suppressing Hh signaling during pancreas formation. In conclusion, Hh signaling inhibits early pancreatic development in the mouse and the exclusion of Shh and Ihh from the pancreatic primordial is critical to normal murine pancreas development.
Although no Shh is expressed in the developing or adult pancreas, low levels of Ihh and Ptch1 mRNA are found with RT-PCR in the murine pancreas from E13.5 onward and in the adult. Ihh , Dhh , and Ptch1 are expressed in the islets of the adult pancreas; Ptch1 is also expressed in the pancreatic ducts. This suggesting Hh signaling in the islets is autocrine. It has been suggested that Hh signaling may act to restrict pancreas growth. Shh −/− mice have a bodyweight of approximately 30% of wild-type embryos at 18.5 d.p.c., their pancreas weight is similar to that in wild-type mice. The Ihh −/− mouse has a body weight of approximately 65% of wild-type mice at this stage of development and its pancreas is similarly 65% of the weight of the wild-type pancreas. It is difficult to infer from these observations if, for example, Shh affects body size but not pancreas size or if Shh restricts pancreas growth as suggested. Almost half of Ihh −/− mice develop an annular pancreas in which the pancreas encircles the duodenum. Histologically, the pancreas appears intact and contains normal islets. Cellular differentiation seems to be more or less normal in both Shh −/− and Ihh −/− mice. Only if the number of endocrine cells is expressed relative to body weight would there appear to be a relative increase in the number of endocrine cells in the pancreas, especially in the Shh −/− mice. The number of endocrine cells/pancreas weight seems a more logical measure. However, this seems only modestly increased in Shh −/− mice (22% increase) whereas the number of endocrine cells/pancreas weight is increased by 75% in the Ihh −/− mice. Since Ihh and not Shh is expressed in the developing pancreas, it seems that Ihh may act as a negative regulator of endocrine cell fate in the developing pancreas. Overactivation of Hh signaling in the pancreas, by deletion of Hhip or through pancreas-specific overexpression of Shh or Ihh , resulted in inhibition of epithelial expansion and loss of endocrine cells, confirming the idea that Hh signaling represses endocrine cell fate.
4.3.2.5
Development of the Liver
Shh is expressed in the liver primordium in the ventral foregut endoderm, where three distinct domains of hepatic progenitor cells have been revealed. These are located in the medial and bilateral regions of the foregut, adjacent to the developing heart. Fgfs derived from the cardiac mesoderm play a major instructive, concentration dependent, role in specification of hepatic cell fate. Besides Fgf signaling also other morphogen pathways such as the bone morphogenetic protein (Bmp)- and Wnt-pathway have been shown to play a role in mechanisms of controlling early development of liver parenchymal cells, see Ref. for a review. However, a role for Hh signaling has not been described and liver development seems to progress normally in both Hh and Gli mutant mice.
4.3.3
Hh Signaling and Development of the Midgut
The midgut will form most of the intestine distal from the common bile duct that derives its blood supply from the superior mesenteric artery: the distal duodenum, the jejunum, ileum, cecum, and the ascending and proximal transverse colon. The intestinal endoderm is initially a pseudostratified cuboidal layer and is converted into a polarized columnar epithelial monolayer in a proximal to distal morphological wave that starts at 15 d.p.c. In contrast to human development, morphogenesis of the murine intestinal epithelium is not complete until three weeks after birth when crypts have developed from intervillus epithelial cells. The formation of villi is initiated in the proximal duodenum at E14 and occurs just preceding cytodifferentiation. The second phase of small intestinal epithelial differentiation takes place after birth. The crypts of Lieberkühn are generated in the small intestine and Paneth cells are formed.
Ihh and Shh are both expressed in the developing midgut and have therefore partially overlapping functions. They regulate endodermal-mesodermal interactions in the developing small intestine as both are produced by endodermal cells and the Hh target genes Ptch1 and Gli1 are strongly expressed in the surrounding mesoderm during development and directly after birth. Ihh is expressed throughout the midgut at 11.5 d.p.c. and is maintained along the length of the midgut throughout development. At E18.5, both Shh and Ihh are expressed at the base of the villi in the small intestine. Initially, Shh is expressed uniformly throughout the midgut, similar to the expression of Ihh , but is downregulated in the small intestine at E14.5, with highest remaining expression in the duodenum. Shh and Ihh mutant mice displayed different phenotypes ( Table 4.1 ). Whereas the duodenum of Shh −/− mice was overgrown with villi, villus size, and precursor cell proliferation were reduced in the Ihh −/− mouse.
Madison et al. studied the phenotype of Villin-Hhip transgenic mice, using a 12.4-kb fragment of the Villin gene to drive expression of Hhip, resulting in inhibition of Hh signaling from E12.5 onwards. The phenotype of the Villin-Hhip mice was more severe than that of the Ihh −/− mice. Villus formation was completely abrogated and regions were observed that had failed to organize into a layer of polarized columnar cells, but instead had remained pseudostratified. This suggests that the main role for Hh signaling in development is villus growth. Lower expression of Villin-Hhip transgene resulted in thickened villi, accumulation of precursor cells, and extensive branching with crypt-like pockets of proliferating cells on the villi, suggesting that Hh signaling restricts the proliferating cells to the intervillus epithelium. Another model in which Hh signaling was disrupted specifically in the epithelium of the developing intestine in the VillinCre-Ihh fl/fl mouse. In these mice, a very similar phenotype was observed as in the Villin-Hhip mice. Proliferation of the epithelial cells was increased, and mice presented with crypt fissioning, villus branching, and ectopic crypt formation. Wnt signaling and stem cell markers were also increased. Furthermore, both models showed reduced number of differentiated cells. Some similarities were observed between the Villin-Hhip and VillinCre-Ihh fl/fl mice on one hand and two mouse models in which Bmp signaling was inhibited, the Villin-Noggin mice and the Bmp receptor 1a knock-out mice, on the other hand. They all showed a similar phenotype of ectopic crypt formation on the villi. In the Villin-Noggin mice, ectopic crypts only became evident 3 weeks after birth, but this could be explained by the fact that a different Villin promoter was used. As Bmp4 is a mesenchymal target of Hh signaling in the small intestine, it is possible that Hh acts upstream of Bmp4 in the control of precursor cell compartmentalization. Recently, Shyer et al. confirmed the predicted role for Shh and Bmp4 in localization of stem cells to the base of intestinal villi. Through differentiation of multiple smooth muscle layers lining the gut, physical boundaries led to folding of the epithelial layer and subsequent formation of villi, thereby altering intestinal morphogen gradients. Consequently, at the tip of each villus, a local maximum of Shh is formed, which drives the mesenchymal expression of Bmp4 leading to restriction of progenitor cell proliferation to the base of the villi.
Hh signaling in the intestine is exclusively paracrine throughout development and adult life. Hh-responsive cells include smooth muscle precursor cells, differentiated smooth muscle cells of the villus core, and muscularis mucosae, myofibroblasts, and pericytes. Both Ihh and Shh null mice show a reduction in the thickness of the circular smooth muscle layer and distinct defects in the development of the enteric nervous system, suggesting that Hh signaling stimulates smooth muscle cell differentiation or proliferation. Kolterud et al. have demonstrated a similar effect in the developing intestine of the Villin-Ihh mice, where increased Hh signaling resulted in smooth muscle cell expansion in the villus cores. Also in the VillinCre-Ihh fl/fl mice disruption of the intestinal and colonic mesenchymal compartment was described with reduced proliferation of pericryptal myofibroblasts. Another finding in the VillinCre-Ihh fl/fl mice was the development of inflammation in the Ihh mutant mice, which will be further discussed below in the section on Hh signaling in the adult intestine ( Section 4.5.3 ).
In conclusion, these data indicate that Hh signaling in the developing intestine is exclusively paracrine and controls the size of the SM cell population in the villus cores of the developing intestine. Loss of Hh signaling results in increased proliferation of epithelial precursor cells and reduced maturation of enterocytes. These effects are via factor(s) in the mesenchyme that remain to be identified.
4.3.4
Hh Signaling and Development of the Hindgut
The hindgut forms the distal transverse colon, descending colon, sigmoid, and anorectum that develop around the inferior mesentery artery. Very low levels of Shh are expressed at the base of the crypts in the colon at 18.5 d.p.c., whereas Ihh mRNA is expressed throughout the epithelial layer from 11.5 d.p.c. until birth. In Ihh −/− mice, the epithelial cells fail to organize into a polarized monolayer, and the epithelium does not organize into crypt-like structures. The colon is partially dilated in Ihh −/− with an abnormally thin wall and lack of neurons in the dilated areas, a phenotype reminiscent of Hirschprung’s disease. These data suggest that signaling by Ihh plays a role in the conversion of the pseudostratified endodermal layer to an epithelial monolayer in the hindgut and regulates the survival of neural crest cells that have migrated into the intestinal mesoderm. Signaling by Shh is important in the distal hindgut as Shh null mice were shown to have anorectal malformations. The anorectum develops with a complex interplay of all three germ layers as it forms at the junction of the endoderm and ectoderm. Ramalho-Santos et al. find an imperforate anus in their Shh −/− mice, the colon ends in a blind sack and does not form a connection with the perineum. Shh −/− mice maintained by Mo et al. display a failure to separate the anorectum from the lower urinary tract, that drain in a common cavity or outlet. Gli2 −/− Gli3 +/− and Gli2 +/− Gli3 −/− compound mutant mice also have a persistent cloaca and a common outlet for the digestive and urogenital tract but a phenotype that is less severe than Shh −/− mice. Gli2 −/− mice have an imperforate anus with a recto-vesical fistula (between the distal intestine and the bladder) and a single urethral opening in the perineum, see also Kim et al. The phenotype of Gli3 −/− mice is very mild with slight anal stenosis. These phenotypes indicate that although Gli3 does play a role in the transduction of the Shh signal to the anorectal mesenchyme, the contribution of Gli2 is much more important similar to the role of Gli signaling in foregut development.
4.3.5
Hh Signaling and the VACTERL Association in Humans
All of the developmental abnormalities observed in Hh-pathway mutant mice are also found in humans. In humans, the nonrandom association of these abnormalities is denoted by the acronym VACTERL. VACTERL stands for vertebral defects (V), anal atresia (A), cardiac defects (C), TE fistula, renal anomalies (R), and limb defects (L). This association is not recognized as a specific syndrome and its different components are found together in varying constellations (see Ref. ) and have been assigned various acronyms (such as VATER, VATERL, etc.). One study that examined 2,493,999 births only reported a single case with combined recognized deformities in all six organs. However, all of these defects have been found in one or more of the several known human and murine mutants of the Hh-pathway by a variety of observers (see Kim et al. for review ). The atresia or stenosis of the esophagus with associated TE fistula that was found in Hh mutant mice is observed in approximately 1 in 2000–5000 live human births. Just as in the Hh mutants, this anomaly can be found in association with other GI malformations in humans such as anal atresia, midgut malrotation, duodenal atresia, and annular pancreas and with malformations of other organs as part of the VACTERL association mentioned above. Even though a strong similarity exists between patients with the VACTRL association and Hh mutant mice, mutations have been scarcely described in patients. Only recently, Yang et al. found a heterozygous mutation located in the N-terminal region of the Gli3 gene in a neonate with esophageal atresia and hemivertebrae. The VACTRL association shows considerable overlap with the Feingold syndrome. Feingold syndrome is caused by germ line mutations in N-myc, which is an established target of Hh signaling. Although no mutations in N-myc have been found in patients with the VACTRL association, this suggests that mutations in the Hh-pathway or important targets of Hh signaling may indeed be its cause.
4.4
Hh Signaling in Homeostasis of the Adult GI Tract
4.4.1
Morphostasis
The molecules that are involved in the regulation of position- dependent cell fate have been found in genetic screens that used developmental models. However, positional information is something that is equally important to many cell types in the adult organism. Although adult tissues seem to be static and stable, many tissues are not, as they have a very high cellular turnover rate and their micro-architecture can be disturbed for example in chronic inflammation. The GI tract is a good example of the importance of positional information in cellular renewal in the adult. At each level of the gut, a common precursor cell produces a module of specialized cell types appropriate for its position along the anterior-posterior axis. These modules display elaborate patterns of differentiation along the radial axis. At some parts of the GI tract, precursor cells generate two functionally different compartments of cells that migrate in opposite directions (e.g., the stomach). Although stability of the resulting complex epithelial micro-architecture is the norm, instability is frequently observed in humans. This instability leads for example to the formation of metaplasia, atrophy, or hyperplasia such as in polyposis. Often these instable epithelial states are at a high risk to progress to GI cancer. This also explains why mutations in morphogenetic pathways, such as the Wnt- and BMP-pathway, can initiate carcinogenesis.
GI epithelial cells undergo a well-controlled maturation process. Precursor cells generate descendants that withdraw from the cell cycle and induce transcription of cell lineage specific proteins. When the cell is fully differentiated, it is shed into the gut lumen or undergoes apoptosis to maintain cellular census. This is a rapid process that is dependent on the cells position along the axis of renewal. It was shown in an important experiment by Hermiston et al. that such processes are regulated in a cell nonautonomous manner and thus controlled by extrinsic regulators of cell fate. The authors performed an elegant experiment in chimeric mice in which some of the intestinal epithelial cells overexpressed the cell-cell adhesion protein E-cadherin. In these mice, the descendants of the transgenic precursor cells migrated considerably slower along the axis of renewal than the progeny of normal precursor cells present in the same chimeric animal. In spite of this difference in the speed of migration, differentiation, and cell death of the epithelial cells occurred at the appropriate moment along the vertical axis and homeostasis was maintained. This experiment demonstrated that differentiation of the epithelial cell is completely dependent on its position along the vertical axis of renewal and therefore extrinsically regulated. Evidence also exist that the proliferative compartment of precursor cells receives negative feedback information from cells in the differentiated compartment. Through such negative regulation, the amount of differentiated cells can regulate the pace of their own replacement. If differentiated cells in the superficial epithelium are lost by damage such a mechanism allows for increased proliferation to guard epithelial integrity. The mediator(s) of this negative feedback loop have not been identified to date but are clearly soluble molecules capable of long-range signaling.
The identification of the extrinsic controls of cell fate is fundamental to the understanding of the regulation epithelial homeostasis and its deregulation. Work from several laboratories has shown that morphogens seem to play an important role as extrinsic regulators of cell fate in the adult and we will review here what is known about the role of Hh signaling in the adult GI tract.
4.4.2
Hh Signaling in Homeostasis of the Adult Esophagus
Up until now very little attention has been paid to the role of Hh signaling in homeostasis of the adult esophagus. The few studies that describe Hh signaling in the normal esophagus show conflicting results. Isohata et al. found that Shh is expressed by the basal layer of the esophageal epithelium and Dhh by the differentiated layer, both by quantitative RT-PCR and immunohistochemistry. However, expression levels for Dhh found by semiquantitative RT-PCR were extremely low and antibodies against Hh homologues are not always reliable. In another study by Wang et al, Hh signaling in the esophagus was examined, but no expression of Shh was detected in normal human esophageal tissue by immunohistochemistry. They found epithelial expression of Shh and mesenchymal Ptch1 expression in Barretťs esophagus. Our group demonstrated that Shh is the only Hh homologue expressed in the mouse esophagus, and that Hh targets are expressed by the basal layer of the esophageal epithelial cells. When activating the Hh-pathway in adult mice, we observed an expansion of the precursor compartment of the basal layer and failed maturation and migration of the epithelial cells. As opposed to the rest of the GI tract, Hh shows to directly target the precursor cell compartment in the esophagus. Further research should be performed to fully define the role of Hh signaling in esophageal homeostasis.
4.4.3
Hh Signaling in Homeostasis of the Adult Stomach
Renewal of gastric epithelial cells is a bidirectional process. The gastric mucosa is a flat surface that contains multiple invaginations or gastric units ( Fig. 4.7 ). Cells that migrate toward the luminal surface secrete the protective soluble mucin MUC5AC and form the gastric pit (the upper part of the unit). Cells that migrate downward form the gastric glands. The proximal glandular stomach (fundus) has small pit regions and large glands, whereas the distal stomach (antrum) has large pit regions and small glands. Fundic glands are composed of parietal cells, which secrete acid, endocrine cells, such as somatostatin-producing D-cells and gastrin-producing G-cells, and mucous neck cells, a cell type with uncertain function and which transdifferentiates when halfway down the gland into zymogenic cells that secrete digestive enzymes. Antral glands are exclusively composed of mucous and endocrine cell types. Barker et al. identified Lgr5-positive stem cells at the base of the glands throughout the pyloric region and limited number of glandular units adjacent to the esophagus and squamous forestomach boundaries. No Lgr5-positive stem cell populations were found in the adult corpus, indicating the existence of other Lgr5-negative stem cell pools. Although at least in part of the gastric glands the stem cells may be located at the bottom of the glandular units, the main proliferating precursor cell compartment is located in the isthmus, somewhere approximately halfway up these units.
In this homeostatic system factors have to control asymmetric cellular differentiation where the appropriate amount of cells adopts either a pit or gland cell fate. The presence of such factors that act as polarizing signals in the gastric units is clear form histopathological observations in patients with hypertrophic gastropathies. These gastropathies include Ménétrier disease and hyperplastic hypersecretory gastropathy (HHG). In Ménétrier disease, transforming growth factor (TGF)α, a ligand for the EGF receptor, is overexpressed leading to excess proliferation and differentiation of pit cells and loss of glandular epithelial cells. In contrast, the hypertrophy in patients with HHG is characterized by an increased mass of gland cells. Shh is broadly expressed by all epithelial cell lineages of the gastric gland. It is highest in parietal cells very close to the precursor cell compartment and gradually diminishes to very low levels as parietal cells migrate to the bottom of the fundic gland. Several studies have now shown that Shh is necessary for progenitor cell differentiation into various gastric cell lineages rather than for proliferation. Dhh is expressed by the adult stomach, but its role is unknown, and Ihh appears to modulate gastric pit cells. Hh signaling in the adult mouse stomach is exclusively paracrine, from epithelium to mesenchyme.
Reduced levels of Shh expression have been observed in gastric atrophy, a condition often caused by chronic inflammation such as in Helicobater pylori infection. Atrophic gastritis is characterized by thinning of the mucosa, progressive loss of glandular cell types, hyperproliferation of the foveolar compartment, and ultimately the development of metaplasia. Furthermore, it is characterized by a pronounced loss of gastric acidity (hypochlorhydria). Mice in which parietal cells are specifically ablated present with loss of gastric gland lineages and expansion of the foveolar compartment. This suggests that parietal cells secrete a factor that regulates the cell fate of the other gastric cell lineages. One of the parietal cell-derived factors that may very well play a role in the development of atrophic gastritis is hydrochloric acid. Loss of gastric acidity results in a similar phenotype as depletion of parietal cells. Another candidate is Shh, which is also expressed by parietal cells. Loss of Shh is correlated with the degree of atrophic gastritis and intestinal metaplasia in H. pylori -infected patients and Mongolian gerbils. Zavros et al. showed that gastric Shh expression and processing depends on circulating gastrin serum levels and gastric acidity. This suggests Shh expression may act in parallel with, or up- or downstream of hydrochloric acid.
During the early stages of infection, Shh is upregulated in inflamed tissues of the stomach. More specifically, when using a mouse model of H. pylori Shh was induced in the parietal cells within 2 days of infection, and expression could be blocked through inhibition of NF-κB signaling. Interestingly, targeted deletion of Hh signaling within the myeloid cell lineage, including macrophages, resulted in an inability for H. pylori to induce macrophage recruitment to the site of infection, implying a role for Shh from the parietal cell to act as a chemoattractant during initiation of gastritis. Nevertheless, Hh signaling is required for the chronic mucosal changes observed in the infected stomach. More recently, Ding et al. showed that Gli is required for metaplastic changes in the gastric corpus during chronic Helicobacter infection. The study demonstrated that Hh signaling plays a significant role in immune cells by regulating their polarization from a pro-inflammatory to an anti-inflammatory state marked by the presence of myeloid- derived suppressor cells (MDSCs). During the course of a chronic Helicobacter infection, Waghray et al. found that Shh expression is progressively lost from parietal cells in mice. More importantly, they found that this loss was not merely a result of loss of gland cell types, but actually preceded it. They treated Shh LacZ reporter mice with omeprazole, a proton pump inhibitor, and Interleukin-1β (IL-1β), one of the key cytokines in H. pylori -associated gastric cancer development, and a potent inhibitor of gastric acid secretion. Both IL-1β and omeprazole lead to a reduction in Shh expression. However, suppression of Shh expression by IL-1β and omeprazole was additive, suggesting that they are mediated at least in part by parallel pathways. Xiao et al. examined the role of Hh signaling in the stomach through generation of a parietal cell-specific knockout for Shh ( HKCre-Shh KO , Table 4.1 ). These mice presented with similar phenotypic changes, such as foveolar hyperplasia and glandular atrophy, which were also previously found in both parietal cell-depleted mice and hypergastrinemic mice. They demonstrated that histamine-stimulated acid secretion is severely compromised and hypergastrinemia develops. They further observed increased Ihh expression and hyperproliferation of the surface epithelium. Octreotide, a somatostatin analog that inhibits the secretion of gastrin, entirely reversed the molecular and morphological changes in the HKCre-Shh KO . This suggested that the effects of loss of Shh on gastric epithelial morphology were to a large degree indirect and the result of hypergastrinemia. A follow-up study by the same group confirmed that mice expressing a parietal cell-specific deletion of Shh on a gastrin-null background displayed no signs of hyperproliferation, which was reversed by infusing gastrin. Interestingly, gastrin-induced proliferation was accompanied by increased expression of Ihh and proliferation was blocked when using the Hh signaling inhibitor vismodegib suggesting the role of Ihh as a regulator of epithelial proliferation in the stomach. A study by El-Zaatari et al. described a mouse that expressed a soluble Hh antagonist in the parietal cells ( HK-sHhip, Table 4.1 ) and confirmed the important role of Shh signaling in parietal cell differentiation and function. They showed that Shh signaling may be regulated directly by established regulators of gastric acid secretion, such as gastrin, actetylcholine, and histamine, which, like Shh, activate signaling pathways through G-protein-coupled receptors. In conclusion, parietal cells secrete factors that affect differentiation of the other gastric cell lineages and are lost in atrophic gastritis. Both hydrochloric acid and Shh seem to be such factors and their regulation seems to be intimately linked.
4.4.4
Hh Signaling in Homeostasis of the Adult Liver
The main cell type of the liver is the parenchymal cell, or hepatocyte, which makes up 80% of hepatic cells. Other cells in the liver include the Kupffer cells, hepatic stellate cells (HSCs), endothelial cells, and lymphocytes. Kupffer cells are the macrophages of the liver and are located within gaps between sinusoidal endothelial cells. The main function of stellate cells is the storage of vitamin A. They reside in the space of Disse, between the hepatocytes. During liver injury, the HSCs, which are normally quiescent, are activated and become myofibroblasts that produce extracellular matrix proteins. They produce most factors that lead to fibrosis. Although much of the work on HSCs has focused on their role in pathologic liver injury, they are also thought to play a role in regeneration of the healthy liver, such as occurs, for example, upon partial hepatectomy. Reconstitution of the liver after partial hepatectomy does not require the recruitment of liver stem or progenitor cells, but involves replication of mature functioning liver cells. It has become clear that in most types of chronic liver injury, replication of mature hepatocytes is inhibited. In this case, recovery relies on expansion and differentiation of progenitor cells. In humans, liver progenitor cells are often described as intermediate hepatobiliary cells. In animal experiments, their rodent counterparts are often called “oval cells,” which is a more general term for liver progenitor cells. The origin of the oval cell precursors is still unclear; however, it has been suggested that oval cells are bi-potential transient amplifying cells derived from stem cells that reside in the biliary tree.
Although healthy mature hepatocytes do not normally express Hh ligands the progenitor cells do. Several studies have shown that HSCs and liver epithelial progenitor cells are capable of both producing and responding to Hh ligands. They rely on Hh for activation and viability and HSCs require Hh signaling for their development into myofibroblasts. In many types of adult liver injury in rodent models Hh-responsive cell populations expand significantly. In livers of bile duct ligated rats, Shh as well as Ihh were found to be increased in peri-portal epithelial cells, representing potential hepatic progenitor cells. Hh signaling declines when recovery is completed. This suggests a role for the Hh-pathway in the reconstruction of damaged adult livers. A recent study indeed showed that the expression of Hh targets increases upon partial hepatectomy in mice and when specifically deleting the Smo receptor from myofibroblasts liver regeneration was impaired. Interestingly, by performing lineage trace experiments, authors demonstrated that myofibroblasts are also able to give rise to epithelial progenitors in order to repopulate the injured liver and that this process can be suppressed when blocking Hh signaling. Also in human adult liver injury, the Hh-pathway is activated. Immunohistochemistry of liver sections from patients with biliary cirrhosis demonstrated increased expression of Ihh, Ptch, and Gli2. Bile duct cells were the predominant source of Ihh, while oval cells, immature duct cells, and myofibroblasts were the Hh-responsive cells.
Hh signaling has also been proposed to play a role in epithelial-mesenchymal transition (EMT) during biliary fibrosis. In many of the cholangiopathies, reactive cholangiocytes are abundantly present in the peri-portal region. They secrete inflammatory cytokines, chemoattractant proteins, and inhibitors of apoptosis. In addition, reactive cholangiocytes lose some of their epithelial phenotypic characteristics. E-cadherin expression is reduced and expression of mesenchymal markers increased. It has been proposed that in fibrotic diseases of the biliary tract, EMT is a potential mechanism for disappearance of bile ductules and development of fibrosis. Omenetti et al. have suggested that upon injury, the HSCs produce Shh. Shh would act on cholangiocytes, which would undergo EMT and migrate into the parenchyma. However, most of their experiments are performed in vitro. Syn et al. performed some in vivo studies and showed that in both mice and humans the Hh-pathway becomes activated in fatty liver injury and that the level of pathway activity correlates with the level of fibrosis and the degree of EMT. However, to provide definite proof that cholangiocytes are able to undergo EMT and become myofibroblasts will require further experiments involving in vivo lineage tracing with cholangiocyte-specific markers.
A critical role for Hh has been described during the development of nonalcoholic fatty liver disease (NAFLD), the most common chronic liver disorder in the Western countries. When hepatic steatosis, a hallmark feature of NAFLD, progresses into more severe steatohepatitis (NASH), liver fibrosis can occur with possible progression into cirrhosis, and primary cancer. Activation of the Hh-pathway was observed in different mouse models of NAFLD, demonstrating both upregulation of Hh ligands and increase in Hh-producing cells. Mice on a NASH-inducing diet developed worse liver disease upon activation of the Hh-pathway, whereas deletion of Smo suppressed an immune response by reducing macrophage activation and proinflammatory cytokine expression in steatotic livers. Matz-Soja et al. suggested a critical function for Hh in liver metabolism by demonstrating lipid accumulation and eventually steatosis upon hepatocyte-specific deletion of Smo. The same group also demonstrated a role for Hh in regulating insulin-like growth factor (IGF) and the regulation of several metabolic genes, including lipid associated factors. In livers of patients with NAFLD, it was recently shown that the expression of Shh is increased and severity of disease correlated with the levels of Shh. The same was observed in a pediatric population in which Hh-pathway activity correlated with fibrosis stage.
In conclusion, the studies discussed above describe a role for Hh signaling in regeneration and fibrosis of the liver. Both Hh ligands and downstream targets are expressed by HSCs and progenitor cells and upregulated upon injury of the liver. Since Hh-pathway activity correlates with disease activity in patients with NAFLD, and deletion of Smo has shown to abrogate liver fibrosis in different rodent models of NASH/NAFDL, the Hh-pathway could be a potential therapeutic target in the treatment of this chronic disease.
4.4.5
Hh Signaling in Homeostasis of the Adult Intestine
The small intestinal epithelium is a homeostatic self-renewing tissue, ordered into crypts and villi. In the mouse, crypt formation is completed in the third postnatal week. Intestinal stem cells are located at the bottom of the crypts. They give rise to transit amplifying cells that proliferate rapidly and differentiate into the four cell lineages of the small intestine. Enterocytes, goblet cells and entero-endocrine cells migrate up from the precursor cell compartment toward the villus whereas Paneth cells stay at the base of the crypts ( Fig. 4.8 ). The organization of the colon is somewhat similar to that of the intestine, with the difference that it lacks villi and Paneth cells. Barker et al. showed that Lgr5 is a marker of intestinal stem cells and that it is expressed by the crypt-based columnar cells. The precursor cells and the differentiated cells are in a dynamic equilibrium in order to balance the rate of proliferation with cell loss at the epithelial surface. This balance depends on the presence of negative feedback loops and Hh signaling has been shown to play a major role in this loop. Whereas during development, both Shh and Ihh are expressed in the intestinal epithelium, conflicting data exist on the expression of Shh in the adult intestine. Shh in the adult mouse intestine was not detected by in situ hybridization, but it has been shown using a Shh Gfp reporter mouse that low levels of Shh may be expressed by rare cells at the crypt base.
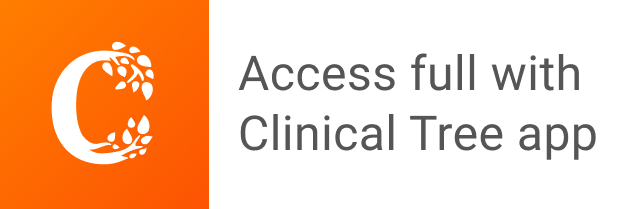