Abstract
Gastrointestinal (GI) tissues express a multitude of growth factors, broadly defined as naturally occurring polypeptides that elicit cellular growth, proliferation, or differentiation following binding to surface membrane receptors. In the GI tract, growth factors perform functions at all stages of life, and play critical roles in development, maintenance, repair and regeneration, pro- and anticancer functions, and immune cell regulation. In this chapter, we discuss the basic physiological processes by which growth factors operate in GI tissue and the signaling underlying their specific roles in health and disease. We review several families of growth factors that have well-defined and significant roles in the GI tract [epidermal growth factor (EGF), transforming growth factor beta (TGF-β), insulin-like growth factor (IGF), hepatocyte growth factor (HGF), and fibroblast growth factor (FGF)] and briefly discuss several other families with emerging roles.
Keywords
Growth factors, EGF, HGF, TGF, IGF, FGF, Intestinal development, Intestinal repair, Intestinal inflammation, Colon cancer
Acknowledgments
The authors would like to thank the contributions of authors of previous editions of this chapter to the development of this text: John A. Barnard and Kirk M. McHugh (4th ed.), and John F. Kuemmerle (5th ed.).
3.1
Introduction
Gastrointestinal (GI) tissues express a multitude of growth factors, broadly defined as naturally occurring polypeptides that elicit cellular growth, proliferation, or differentiation following binding to surface membrane receptors. The growth factor field arguably took root in the 1950s and 1960s with the identification of a number of soluble biological activities necessary to drive cell division. Over subsequent decades, many additional molecules were identified by efforts to find mediators involved in the transformation of normal cells to tumor cells (which characteristically display rapid proliferation and enhanced survival). These early findings laid the groundwork for a wealth of subsequent studies identifying mechanisms of growth factor secretion, receptors, modes of signaling, downstream pathways, and additional ligands that cosignal within each family. In the GI tract, growth factors perform functions at all stages of life and play critical roles in development, maintenance, repair and regeneration, pro- and anticancer functions, and immune cell regulation. In this chapter, we review several families of growth factors that have well defined and significant roles in the GI tract: epidermal growth factor (EGF), transforming growth factor beta (TGF-β), insulin-like growth factor (IGF), hepatocyte growth factor (HGF), and fibroblast growth factor (FGF). We will also briefly discuss several other families with less well defined but emerging roles in the GI tract. An overview of these growth factors, their principal receptors, and targets of actions are listed in Table 3.1 .
Growth Factor Family | Receptors | Ligands | Target Cell Types |
---|---|---|---|
Epidermal growth factor | EGFR/ErbB1 | EGF, TGF-α, NRG1-4, HB-EGF, amphiregulin, betacellulin, epiregulin, epigen | Epithelium |
ErbB2 | Endothelium | ||
ErbB3 | Immune | ||
ErbB4 | |||
Transforming growth factor-β | TβR-I | TGF-β, BMP2-7, activin, inhibin, nodal | Epithelium |
TβR-II | Endothelium | ||
Immune | |||
Insulin-like growth factor | IGFR1 | IGF1 | Epithelium |
IGFR2 | IGF2 | Endothelium | |
Hepatocyte growth factor | c-Met | HGF | Epithelium |
Coreceptors (CD44) | Endothelium | ||
Mesenchyme | |||
Immune | |||
Fibroblast growth factor | FGFR1-4 | FGF1, 2, 4, 7, 9, 10, 15/19, 18, 21, 20 (in cancer), 23 | Epithelium |
Coreceptors (α/β-klotho) | Mesenchyme | ||
Trefoil factor | CXCR4 | TFF1, TFF2, TFF3 | Epithelium |
Unidentified others | Immune | ||
Hedgehog | Ptch1-2 | Shh, Ihh, Dhh | Epithelium |
Immune |
The naming convention of peptide growth factors typically has relied upon the molecule’s apparent function when initially discovered. While this seems straightforward, subsequent research has invariably identified additional and sometimes even contradictory roles for these peptides; a growth factor may elicit a wholly distinct function than what the name would suggest depending on tissue location and context of expression. For instance, EGF has pleiotropic actions in many tissues including but not limited to the epidermis, and some EGF family members can have seemingly “nongrowth” activities such as promoting apoptosis or regulating ion transport. Transforming growth factor (TGF)-β has roles outside of transformation of normal cells, including wound healing, differentiation, and fibrosis, and in many settings actually acts as a tumor suppressor. Furthermore, though TGF-β and TGF-α have names that would suggest close relatives, they in fact belong to entirely different families of growth factors—TGF-β is the prototypic member of a whole family (the TGF-β superfamily), while TGF-α is an EGF-like molecule. Thus, the reader is cautioned against drawing too many conclusions regarding a peptide’s function from its name.
Although other classes of proteins such as cytokines/chemokines and peptide hormones can stimulate cellular growth; in this chapter, we will focus strictly on the traditional peptide growth factors. Cytokines can impact cell growth as a secondary outcome but in general tend to be immunomodulatory in function. Hormones are generated in glandular tissue and are typically secreted into circulation thus allowing for long-range actions. In contrast, peptide growth factors are sourced from a variety of cell types and tissues and are typically categorized as having autocrine, paracrine, or juxtacrine actions ( Fig. 3.1 ). Cytokines, chemokines, hormones, and similar molecules are covered in other chapters.

Growth factor signaling in the GI tract is tightly regulated, in part due to the varied sources and targets of these peptides. It is important to keep in mind the ligand source and receptor location to understand fully differences in signaling outcomes. Growth factors are produced in a variety of tissues and histological strata including the epithelium, the subepithelium, exogenous sources such as breastmilk, and anatomically distinct sources (i.e., luminal EGF secreted from salivary and Brunner’s glands ). In some instances, notably with TGF-β and IGF, the source of growth factor can also be from recruited immune cells. Signaling specificity is supported by restricted or differential receptor expression on different cell types, including epithelial cells, endothelial cells, mesenchyme/fibroblasts, smooth muscle cells, and immune cells, among others. Regulated induction or suppression of ligand and receptor levels (e.g., Refs. ) can alter the responses of specific cell types or the overall tissue as well. Substantial work on each family of molecules in the past half century has revealed specific signaling pathways and alterations in intracellular activity that are stimulated by these growth factors. As some of these signaling pathways and intracellular events are shared, it is important to keep in mind the source(s) of ligands as well as expression patterns of the target receptors in understanding the context-dependent roles and specific outcomes.
Signaling initiated by growth factors occurs through a sequence involving ligand release (or presentation of tethered ligand) and extracellular binding to receptor; recognition of this event is then transmitted to intracellular signals through a variety of methods (predominantly, but not exclusively, kinase activity). Generally, a number of ligands can act through the same receptor. This initially was interpreted as a functional redundancy, but in the past few decades, it has become clear that while there is substantial overlap between shared ligands, conformational and contextual (including spatial and kinetic) differences in responses also come into play that finely tune the output. Thus, availability of a panel of highly similar but not quite identical ligands allows for greater flexibility in control of cell behavior. A significant amount of effort has gone into clarifying these aspects of growth factors in the GI tract, resulting in an evolving understanding of the physiological roles of closely related growth factors, but to a large extent this aspect of the field is still in its infancy. The spatial distinctions, differences in expression, and compartmentalization of related growth factors that share common receptors should be kept in mind when interpreting how the growth factor may function in vivo. These differences and any known implications for physiological function will be discussed within each subsection.
The groundwork laid in understanding the activity and function of peptide growth factors in cell culture was instrumental for determining the role of many of these molecules in maintenance of tissue homeostasis. Furthermore, understanding their activity has allowed us to recognize cases where inappropriate gain- or loss-of-function is involved in the development of disease. At the same time, understanding the basic biology and biochemistry of these molecules has allowed for harnessing this knowledge to advance therapeutics and disease treatment. For instance, in mouse models, a number of growth factors are dispensable for normal homeostatic maintenance/tissue turnover but absolutely required for appropriate and efficient response to injury and inflammation. In other cases, overexpression or lack of expression of growth factor signaling can result in profound baseline GI disturbances, implicating specific factors in tissue homeostasis, and in several cases are associated with disease states such as cancer.
3.2
Epidermal Growth Factor (EGF) Family
3.2.1
Introduction
The EGF family is a large group of peptide growth factors that, through activating one or more of the ErbB receptor tyrosine kinases ( Fig. 3.2 ), influence cell proliferation, differentiation, migration, function, and survival in the GI tract. EGF, the prototypic member of this family, was initially described in 1962 as a protein in mouse submaxillary gland extracts that promoted premature eye opening and incisor eruption in newborn mice. Decades of subsequent study on this “tooth-lid factor” have demonstrated critical roles for EGF and its relatives in most cell types in the body. The EGF family has expanded to include additional peptides including TGF-α, heparin-binding EGF-like growth factor (HB-EGF), heregulins/neuregulins (HRG/NRG), amphiregulin (AR), betacellulin (BTC), epiregulin (EPR), and epigen (for review, see Refs. ). These molecules share substantial sequence homology and, with varying degrees of affinity, the same receptors. Thus, they evoke overlapping but not completely identical biological activities, with differential receptor binding providing a first layer of explanation for the differences.

Each EGF family member is synthesized as an integral membrane protein with functionally distinct cytoplasmic, transmembrane, and extracellular domains and typically harbor extracellular proteolytic cleavage sites for processing by distinct members of the “a disintegrin and metalloproteinase” (ADAM) family of sheddases ( Fig. 3.3 ). Following cleavage and processing, EGF-like peptides bind and activate EGFR, ErbB3, and ErbB4. A fourth receptor, ErbB2, is involved in transducing signals from these ligands but does not appear to bind them directly. The receptors were named ErbBs due to their close homology with the v-erbB oncogene encoded by the avian erythroblastosis virus. Alternatively, they are also known as human epidermal growth factor receptor 1-4 (HER1-4). The choice of naming of these receptors in the literature is largely a factor of historical convention within the field of study—for instance, the breast cancer field typically relies on using the HER naming strategy, whereas the GI field generally uses the EGFR/ErbB names. Regardless of the name, upon ligand binding, the receptors are typically stabilized in homodimers (two of the same receptor) or heterodimers (two different ErbBs), leading to increased tyrosine kinase activity, transphosphorylation of the kinase cytoplasmic domain, and presentation of tyrosine-phosphorylated docking sites for downstream signaling adapters and substrates.

Signaling through ErbB/HER receptors is complex, allowing for fine regulation of cellular outcome. A common component of ligand-induced signaling that is recurrent in growth factor biology is receptor dimerization. Within the EGFR/ErbB family, the number of ligands (each with individual affinity profiles for the different receptors), combined with the number of potential receptor dimers, allows for substantial diversity in cellular outcomes. For example, ErbB3 has very low kinase activity, while ErbB2 has no known ligand and typically functions in heterodimers. Furthermore, each receptor has a distinct pattern of c-terminal docking sites for downstream signaling, and different ligands can preferentially stabilize different dimer combinations to elicit different cellular effects. Thus, while signaling through these receptors can clearly have profound effects on cell growth and wound repair in the gut, the family can be “reused” with slight variations to control other cellular functions such as ion transport and differentiation.
3.2.2
Epidermal Growth Factor (EGF)
The study of EGF over the past half century has been foundational for our modern day understanding of classical ligand-receptor activation and signaling paradigms within the field of molecular and cellular biology. The importance of this body of work was underscored in 1987 when the Nobel Prize was awarded to Stanley Cohen and Rita Levi-Montalcini for the discovery of EGF and nerve growth factor. In the GI tract, EGF is produced in multiple compartments including the salivary glands, intestine, stomach, esophagus, and accessory organs (pancreas and liver). EGF elicits its classical mitogenic effects by binding EGFR on the cell surface, leading to receptor dimerization and tyrosine kinase activity. It is mainly exclusive to EGFR homodimers and EGFR/ErbB2 heterodimers in terms of binding affinity, though low binding to ErbB2/4 dimers has also been reported. Heterodimer activity can be important as asymmetric dimers not only have a broader palette of intracellular docking sites but can also be “biased” toward different ligands and have different internalization-downregulation profiles after activation. Following receptor binding and dimerization, phosphorylation of specific c-terminal tyrosine residues activates signals through a wide array of pathways including ERK MAPK, p38 MAPK, JNK, and PI 3-kinase, resulting in numerous context-dependent cellular outcomes including altered DNA synthesis, intracellular calcium influx, glycolysis, and selective regulation of gene and protein expression.
Sources of secreted EGF in the GI tract include the submaxillary glands and the Brunner’s glands of the duodenum. Both of these sources result in luminal secretion. This is important to note because EGFR is expressed predominantly on the basolateral surface of the intestinal epithelium. Thus, utilizing the luminal pool of EGF requires that the ligand somehow cross the barrier. This originally led to the hypothesis that EGF is a luminal surveillance factor, poised to react to injured mucosa (where the barrier is naturally broken) by stimulating cellular proliferation, migration, and repair. In adults, transepithelial migration of EGF does not readily occur; therefore, luminal EGF is primarily available to pass the barrier when it is breached to promote epithelial wound repair as well as interact with other cells expressing EGFR such as immune cells. Alternatively, there is some evidence for EGFR redistribution to the apical face of enterocytes after injury. However, EGF can also be induced in subepithelial myofibroblasts, and it is released by the Paneth cells near the small intestinal crypt base. This may establish a gradient of available EGF along the crypt-villus (crypt-surface mucosa in the colon) axis in homeostatic turnover, which is then disrupted by injury. Furthermore, the expression of EGF in ulcer associated epithelial cell lineages suggests it can be produced locally throughout the intestine in response to chronic damage. Interestingly, another route through which EGF can access the GI tract in early life is through breast-feeding, as mother’s milk has been shown to be a major source. This suggests EGF has a role in development and maintenance of the intestinal epithelium in this sensitive early period of life, consistent with pig models of development in which weaning-associated villus atrophy can be prevented, and growth curves enhanced, by supplying EGF.
Among other functions in the intestinal epithelium, EGF stimulates cell migration and restitution, increases proliferation, delays intestinal cell shedding, and modulates nutrient and ion transport. EGF infusion or administration results in elevated epithelial cell proliferation, increased small intestinal villi size, and increased intestinal diameter. Additionally, it has been shown to have important roles in the regulation of the intestinal stem cell niche. This role of EGF in maintaining the stem cell niche is highlighted by its requirement in cultures of self-organizing 3D cultures of intestinal mini-guts, known as enteroids, and gastric organoids.
The pleiotropic responses to EGF signaling are of course tightly regulated, both at the cellular and tissue levels. At the most basic level, EGFR activation at the cell surface triggers receptor endocytosis and either recycling or destruction of the receptor depending on strength and duration of signal (for review, see Ref. ); interruption of the internalization-ubiquitinylation-degradation sequence can have profound effects on cellular growth. Additional physiologic feedback loops on ligand expression and availability present another level of control. For example, in the stomach, EGF is digested by gastric acid to less active forms, while EGF also serves to inhibit gastric acid secretion. In the intestine, EGF can induce other ErbB ligands and can itself be negatively regulated by inflammatory cytokines. Multiple reciprocal feedback mechanisms allow for fine tuning of the signaling response and attendant proliferation, wound healing, and other homeostatic activities.
Many examples of enhanced EGF expression or signaling have been demonstrated following an insult to the GI mucosa or in context of disease. In general, these studies identify induction as a cytoprotective response contributing to restoration of health and homeostasis. In rat studies, intraperitoneal injection of EGF (which would have basolateral access to EGFR) conferred protection of the small intestinal mucosa against methotrexate-induced injury. In liver, following partial hepatectomy and in cirrhosis, EGF expression is induced and important for hepatic regeneration. In chronic intestinal inflammation, EGF expression is elevated in the ulcer associated cell lineages, which may contribute to epithelial restitution and repair. Under pathological conditions, EGF has been shown to attenuate intestinal damage and enhance mucosal healing. Animal model results showing that EGFR gain- or loss-of-function are protective or sensitizing to intestinal injury, respectively, strongly support a role for EGF in driving resolution of colitis. In fact, in a limited clinical trial with mild-to-moderate ulcerative colitis patients, the Playford group demonstrated an impressive 83% response rate, including enhanced mucosal healing, to EGF when given as an adjunct to mesalamine.
In addition to studies of adult disease, work with administration to neonatal rodent pups has suggested that proper EGF signaling may be important in the prevention of neonatal intestinal inflammatory disease, such as necrotizing enterocolitis (NEC). Animals receiving EGF are protected from the formula feed-hypoxia model of NEC. This is presumably through suppressing epithelial apoptosis and encouraging rapid and efficient healing of epithelial damage, though secondary actions by other environmental factors such as bile acid pools may also contribute. These findings are also supported by some limited NEC work in human trials and have led to the hypothesis that lack of this growth factor (normally available in expressed breast milk ) may contribute to the increased NEC risk associated with formula feeding. Altogether, there is a substantial body of evidence suggesting that EGF could be useful therapeutically for promoting mucosal repair.
Despite the potential demonstrated in preclinical work, concerns over possible tumorigenic effects have limited exploration of EGF as a therapeutic agent (for discussion, see Ref. ). While EGF can drive wound repair and recovery of homeostasis, unregulated signaling through its receptor EGFR also plays a role in GI neoplasias. ErbB receptor tyrosine kinases have shown some promise as therapeutic targets in a variety of epithelial cancers including in colon. Altered EGF expression has been described in a number of tumors including pancreatic, esophageal, gastric, hepatic, and colorectal cancers. Furthermore, the potential for EGF to contribute to GI neoplasia has been suggested by mouse models, for example, the development of hepatocellular neoplasia in transgenic mice that overexpress secretable EGF. However, it should be noted that mutation, amplification, or forced overexpression represent radically different mechanisms than activation of the endogenous receptor with its normal ligand, even if the ligand is given therapeutically (acutely, instead of constitutive overexpression). Studies with mice deficient in EGFR signaling indicate that endogenous signaling actually acts as a tumor suppressor in some circumstances. As with many other regulatory peptides, EGF-driven signaling likely sits in a “Goldilocks” zone, with either excessive or insufficient activity leading to disease.
Loss-of-function studies suggest a functional redundancy for EGF exists in the intestinal tract. For example, administration of exogenous peptide is trophic for the GI tract and protects against intestinal injury, whereas EGF knockout mice have neither an overt intestinal phenotype nor exaggerated sensitivity to acute DSS colitis (though chronic colitis has not been well-described in this context). In contrast, the EGFR wav5 mutation, which encodes a receptor with very low activity, does predispose to and worsen colitis. This apparent contradiction is generally thought to be the result of ligand redundancy and/or compensation, though it is also clear from cell culture studies that individual ligands elicit distinct molecular and cellular responses. In fact, to achieve a histologically notable phenotype (in this case, spontaneous duodenal ulceration) in the absence of additional challenge (e.g., nonsteroidal antiinflammatory drug-mediated injury, cytokine injection), Troyer and colleagues deleted three different ligands —EGF, amphiregulin, and TGF-α. It is unclear whether the effects of this triple deletion are exactly equivalent to total loss of EGFR/ErbB1 signaling in the epithelium, as whole body knockout of the receptor is lethal in early life, though the GI tract is definitely perturbed in EGFR −/− pups. Furthermore, data from conditional knockout mice have not yet been reported. Overall, however, targeting ErbB receptors directly tends to have a greater physiological impact than deletion of limited sets of ligands.
Another complication with interpreting the results from ligand knockouts is recent data showing differential involvement for EGF signaling in different cell types; for example, while it is clear that EGFR activation in epithelial cells is protective, in macrophages it can promote inflammatory responses and contribute to colitis, as EGFR deletion from cells of the myeloid lineage (including macrophages) reduced disease in the murine DSS model. Similarly, in Helicobacter -induced gastritis, lack of macrophage-specific EGFR resulted in decreased gastric inflammation, though this was accompanied by increased bacterial load. These results demonstrate a role for EGF signaling in GI physiology and disease beyond its traditional role as a mitogenic factor. Further studies of the cell type-specific effects of EGF (and other ligands), and comparisons between the role of endogenous (and thus physiologically localized) versus exogenous, therapeutic application are warranted.
3.2.3
Transforming Growth Factor-Alpha (TGF-α)
Next to EGF, the most extensively studied EGF-family ligand in the GI tract is TGF-α. This molecule was initially discovered in the 1980s in screens designed to uncover factors involved in transformation of normal cells to malignant cells. In particular, these efforts were meant to identify factors that could be produced by the cells themselves that have self-regulating autocrine activity. These screens using assays of growth in soft agar as a measure of tumorigenicity identified TGF-α and TGF-β as cooperative drivers of transformation. As noted above, despite their names TGF-α shares sequence homology with EGF rather than TGF-β, competes with EGF for binding sites, and shares many biological activities of EGF owing in part to its affinity and signaling through the same receptor, EGFR.
TGF-α is readily expressed in the GI tract in fetal and adult stages and found as early as 10 weeks into gestation in humans that persists throughout life. Animal studies also suggest that GI expression of TGF-α may decrease with age. Expression is detectable in most epithelial cells of the GI tract at low levels including stomach, esophagus, small, and large intestines. In the stomach, levels are highest in parietal cells. In the esophagus and intestine, it is predominantly found in differentiated epithelial cell populations. In a similar manner to EGF and other growth factors, TGF-α is proteolytically cleaved from its membrane-bound proprotein by ADAM metalloprotease sheddases into the active form.
At first glance, substantial redundancy between TGF-α and EGF might be expected, and indeed in a number of tissues, the mature TGF-α plays similar roles to EGF in terms of cellular proliferation and differentiation. However, these molecules display clear differences in regulation of expression, localization/compartmentalization, EGFR binding, and biochemical effects on the receptor. For instance, EGF is secreted from distinct sites of the GI tract, whereas TGF-α is more broadly expressed, thus segregating the effects of these two growth factors based on location. TGF-α tends to be available primarily basolaterally, whereas the major portion of EGF seems to be apical. Furthermore, TGF-α and EGF bind EGFR with different kinetics ; this contributes to different profiles of downstream effector binding as well as different endocytic trafficking of ligand- receptor complexes leading to either EGFR recycling or destruction.
In vivo studies have shown that active TGF-α has trophic effects on the GI epithelium and stimulates epithelial growth, enhanced restitution, and cellular proliferation. It is important to note that TGF-α is sorted largely to the basolateral compartment. As its receptor, EGFR is also basolaterally located, it has been suggested that in contrast to EGF juxtacrine or paracrine TGF-α activity may be important for homeostatic regulation of the GI tract. Highlighting this difference in compartmentalization, TGF-α administered enterally shows no significant effects on proliferation, demonstrating a lack of functional redundancy between EGF and TGF-α in this tissue. Alternatively, TGF-α administered parenterally results in stimulation of GI growth and cell proliferation. Furthermore, TGF-α overexpressing mice show hyperplastic gastric mucosa. Knockout mice for this ligand or animals transgenically overexpressing it display increased susceptibility to, or protection from, DSS colitis, respectively. Thus, TGF-α is clearly an essential part of the injury response in the intestine.
As with EGF, TGF-α also plays a role in hepatocyte growth and liver regeneration. In the partial hepatectomy model, TGF-α is induced in liver and circulation and correlates with increased DNA synthesis in the regenerating liver. Interestingly, TGF-α is not required for regeneration as mice deficient in this ligand are still able to exhibit hepatocyte proliferation. This suggests EGF or other EGFR-signaling molecules compensate and restore liver mass in these settings. It is interesting to note, however, that TGF-α overexpressing animals have increased liver mass and elevated DNA synthesis and hepatocyte proliferation, demonstrating the significant effect of TGF-α on liver growth.
TGF-α also has a variety of nonmitogenic roles in the GI tract. Like EGF, it limits gastric acid secretion, but is also rendered less active by gastric acid in the stomach demonstrating a regulatory loop in its activity. TGF-α has also been shown to modulate ion and nutrient transport, stimulate mucin secretion, and mesenteric and mucosal blood flow and promote smooth muscle contraction. Together, these roles appear to promote tissue maintenance and repair following GI injury.
Not surprisingly in light of how TGF-α was discovered, this ligand is deregulated in many GI cancers, including esophagus, stomach, and colon. Several lines of evidence suggest the overexpression of TGF-α is associated with severity of tumorigenicity. For instance, TGF-α expression positively correlates with tumor severity and is expressed in serum in the case of GI tumors, including gastric, pancreatic, and colon. Furthermore, activation of the TGF-α receptor, EGFR, is increased in GI cancer opening the field for therapeutic intervention using several FDA-approved EGFR inhibitors that could act in part by limiting the action of TGF-α (gefitinib, erlotinib, cetuximab, panitumumab, and lapitinib). Efforts to identify patients who would be most responsive to specific treatments or chemotherapeutic agents are still underway.
3.2.4
Neuregulin (NRG)
NRG growth factors (also known as heregulins, HRG) are a subclass of EGF-like molecules that were initially identified in the 1990s in screens for ErbB3 and ErbB4 ligands. Since this time, the study of NRGs has expanded dramatically, and roles for this family of growth factors have been identified in cardiac development, neuronal function, metabolism, epithelial cell development and survival, and immune cell activation. The NRGs harbor mitogenic roles including growth, differentiation, and survival of epithelial, glial, and muscle cells in vitro.
Mammals harbor four neuregulin genes (NRG1-4). NRG transcripts are readily detected in the liver, stomach, small intestine, and colon. Substantial splice variation as well as multiple transcriptional start sites per gene allow for production of a wide array of distinct molecules, including for example up to 15 different NRG1 peptides and 4 or more NRG4s. The functional significance of the different variants of each gene product have, for the most part, not yet been explored. The most widely studied ligand, NRG1β (a.k.a. HRG1β), is expressed throughout the GI tract including esophagus, stomach, intestine, and colon. Aside from a few recent papers on NRG4, most of what is known about neuregulins in the gut is based on work with NRG1β. It should also be noted that the majority of functional studies, especially in cell culture or administration to animals, have used soluble extracellular domain peptides (i.e., the “business end” of the molecule). Thus, many of these findings will be cross-applicable not only to a number of different isoforms but also leave out entirely the impact of regulatory effects on cleavage/secretion or potential juxtacrine signaling from tethered ligand.
The majority of NRGs are synthesized as preproteins inserted in the plasma membrane, thus requiring proteolytic cleavage by ADAM sheddases. Notable exceptions include the B-type variants of NRG4, which are missing the transmembrane domain and appear to be expressed in the cytoplasm, with as-yet unknown function. Since the 1990s, experimental evidence has uncovered the affinity of the shed ligands for each of their various ErbB receptors. NRG1 and NRG2 bind and activate both ErbB3 and ErbB4 (with some splice variant-driven specificity between α and β variants preferring ErbB3 and ErbB4, respectively), while NRG3 and NRG4 exclusively bind ErbB4, at least in cell culture experiments.
Functionally, NRGs and their receptors in the intestine have been shown to have roles in cytoprotection, regulation of chloride secretion, development of the enteric nervous system, and epithelial secretory cell differentiation. NRG4, in particular, has antiapoptotic effects on the intestinal epithelium ; on the other hand, it can elicit cellular death responses in other cell types such as inflammatory macrophages. The ability to kill inflammatory macrophages while protecting the intestinal epithelium from cytokine-induced apoptosis is the likely explanation for the protective effects of NRG4 in colitis models. Additionally, ErbB4 (the NRG4 receptor) has been associated with hypoxia signaling and can stabilize hypoxia-induced factor-1α, which tends to be protective in the gut. ErbB4 stabilization of hypoxia-induced factor-1α was associated with altered regulation of cellular metabolism, which together with studies showing NRG4 has a protective effect versus obesity-associated inflammation suggests a likely role for NRGs in the link between hypoxia and metabolism, which may be very important in the gut.
Dysregulation of NRG signaling has been linked to GI disease development. Whole-exome sequencing revealed that NRG1 is frequently mutated in IBD-associated colorectal cancer. Polymorphisms or deletions of this gene as well as of NRG3 are associated with Hirschsprung’s disease. NRG1 and 2 are both decreased in KRAS-mutant colon cancers. NRG1 and NRG4 expressions are suppressed in total parenteral nutrition-associated intestinal inflammation (where excessive intestinal epithelial apoptosis is observed), but can be rescued by EGF administration. NRG4 expression is decreased in human inflammatory bowel disease and rodent models of colitis. Interestingly, restoration of NRG4 ameliorates disease parameters in several animal models of colitis, suggesting harnessing neuregulin signaling may be a beneficial therapeutic target in the GI tract.
3.2.5
Heparin-Binding Epidermal Growth Factor-Like Growth Factor (HB-EGF)
Heparin-binding epidermal growth factor-like (HB-EGF) growth factor was initially identified as a mitogenic factor present in the conditioned media of a tumor macrophage cell line. Its expression is induced by TNF and oxidative stress, among other proliferative stimuli. Like other EGF-related peptides, HB-EGF is synthesized as a transmembrane protein and proteolytically cleaved by ADAM sheddases to generate its mature, active soluble form. The dominant activities of HB-EGF are stimulating cell proliferation, survival, and cell motility. HB-EGF binds EGFR and ErbB4 to induce phosphorylation. Limited evidence exists supporting different functions of HB-EGF between these two receptors. For example, in cells expressing ErbB4 but not EGFR, the effect of this ligand is chemotactic as opposed to proliferative.
HB-EGF has been identified in gastric parietal cells, but its intrinsic expression elsewhere in the GI tract under homeostatic conditions is low. Exogenous sources of HB-EGF include recruited macrophages and breast milk. In the intestine, HB-EGF is rapidly induced in response to acute injury, where it has been shown to protect against hypoxic injury and may play a role in wound healing. In intestinal, kidney, and liver epithelial cells, enforced expression of or treatment with HB-EGF prevents cell death in response to apoptotic stimuli (such as TNF), demonstrating its role as an epithelial survival factor. These findings suggest growth factor signaling elicited by a recruited cell type or source (i.e., macrophages, breast milk) may be an important role in the protection of GI epithelium following damage. In this regard, HB-EGF has been studied fairly extensively for activity against NEC. Exogenous HB-EGF treatment is effective in preventing incidence and severity of formula feed/hypoxia-induced NEC in rat pups, as is overexpression in transgenic mice ; conversely, knockout animals were more susceptible to experimental NEC. Protection with HB-EGF is accompanied by improved barrier function, reduced epithelial apoptosis, and a reduction in damage to intestinal microcirculation. Interestingly, HB-EGF also altered the balance of macrophage polarization in the gut to a more repair-directed (so-called “M2” population) phenotype in this model, suggesting that similar to NRG4 and EGF, HB-EGF has important effects on the innate immune cells during inflammation, though each ligand seems to have a very different mechanism of action in these cells.
3.2.6
Other EGF-Like Growth Factors: Amphiregulin, Betacellulin, Epiregulin, Epigen
Amphiregulin (AR) was first described in 1988, and is named for its ability to stimulate or inhibit cell growth depending on the cell type under study. AR is expressed in the GI tract in normal and neoplastic cells and binds exclusively to EGFR. At baseline, it is expressed at low levels, primarily in the small intestinal epithelium and surface epithelium of the colon. Levels in both the epithelium and the mesenchyme increase in inflammatory bowel disease and colon cancers. Studies have shown AR stimulates epithelial cell growth and may promote tumorigenesis via an autocrine loop ; it may also be packaged in exosomes and stimulate tumor growth through this mechanism. In vivo studies of AR-null mice show no obvious baseline defects in the GI tract without additional challenge, supporting a normal physiological role primarily involving response to injury or inflammation rather than homeostatic turnover.
Similar to amphiregulin, epiregulin (EPR) can have either stimulatory or inhibitory effects on the growth of cells depending on context. This ligand, first described in 1995, is produced both by stromal fibroblasts and epithelial cells, and like AR is induced by challenge. EPR appears to be important for repair, as deletion results in increased susceptibility to DSS-induced colitis. However, EPR knockouts show no overt developmental defects, suggesting this ligand is also more important in response to injury than homeostasis. It is dispensable for intestinal tumorigenesis, but can contribute to tumor growth and to expansion of Lgr5 + stem cells during regeneration and carcinogenesis.
Betacellulin (BTC) was first identified in 1993 from a mouse insulinoma cell line and appears to be expressed in pancreas and throughout the epithelium of the small intestine and colon. It is also a component of expressed milk, at least in cows. Relative to EGF, it signals through distinct patterns of EGFR phosphorylation suggesting distinct signaling outcomes. In fact, a recent analysis clustering EGFR ligands based on recruitment (and timing) of downstream effectors grouped EGF, amphiregulin, and EPR together, with BTC, TGFα, and epigen forming a second cluster and HB-EGF standing unique. Furthermore, like HB-EGF BTC can bind ErbB4 as well as EGFR, adding additional complexity to its potential function. BTC stimulates crypt cell proliferation and increases intestinal size, dependent on EGFR signaling, in mice, and may have antiapoptotic effects on intestinal epithelial cells. Furthermore, in a transgenic overexpression system, BTC promoted tumor development (multiplicity) in Apc min mice. The explicit role for this ligand in normal physiology or whether it is required for intestinal tumorigenesis remains unclear.
The intestinal function for epigen, the most recently identified ligand in this group has not to date been reported. EPGN expression in the small bowel and colon is supported by online datasets in the Gene Expression Omnibus ( https://www-ncbi-nlm-nih-gov.easyaccess2.lib.cuhk.edu.hk/geo/ ). Thus, it likely has as-yet-undescribed roles in intestinal physiology.
All or nearly all of the ligands that bind ErbB family receptor tyrosine kinases are represented in the GI tract. While there appears to be substantial overlap in the function of these ligands, detailed analyses have demonstrated both shared and distinct roles in GI physiology. In-depth studies focused on understanding the individual regulation, biochemistry, and function of these ligands have the potential to uncover new approaches to leveraging signaling driven by these ligands for clinical use in GI disorders.
3.3
Transforming Growth Factor-Beta (TGF-β) Family
3.3.1
Introduction
As previously mentioned, TGFβ was initially isolated during a screen in the early 1980s for factors that transform normal cells. It was known at the time that malignant cells grew without requirement for the same level of exogenous growth factors as normal cells, and thus uncovering what endogenous growth factors maintained their growth (potentially through an autocrine loop) was of high interest. Treatment of normal fibroblastic cells suspended in soft agar with conditioned media from transformed mouse fibroblasts stimulated growth of these colonies, and extensive work went into characterizing factors in the transformed cell media. These early screens (which also identified TGF-α) implicated TGF-β as a secreted cell-transforming factor. TGF-β has since evolved into the prototype molecule of a new superfamily with pleotropic effects, including tumor suppression . Other members of the TGF-β superfamily now include bone morphogenic proteins (BMPs), activins and inhibins, nodal, and others. Early studies showing high TGF-β concentration within mitochondria of liver and cardiac muscle suggested that it may directly regulate cell metabolism to control mitogenesis. Significant early work in the 1990s revealed a role of TGF-β signaling in many areas of GI development and homeostasis.
TGF β-related peptides are synthesized as precursor propeptides and processed to release biologically active protein. Canonical signaling occurs through heterodimeric receptors, each of which is made up of a type I and a type II TGF-β receptor (TβR) peptides ( Fig. 3.4 ). TGF-β binding to TβRII allows stabilization of a complex with TβRI and phosphorylation of TβRI by TβRII. TβRI subsequently phosphorylates SMAD (mammalian homologs of Drosophila mothers against decapentaplegic and Caenorhabditis elegans SMA) proteins (most notably SMAD2 and 3), which then form multiple SMAD complexes, accumulate in the nucleus, and regulate transcription.

3.3.2
Transforming Growth Factor-Beta (TGF-β)
Three genes expressing canonical TGF-β isoforms have been described, named TGF-β1-3. In the gut, TGF-β expression has been well characterized in the small and large intestine within the lamina propria and epithelial cells. Although other members are present, TGF-β1 is the most broadly studied ligand in the GI tract to date. It should be noted that while the studies forming the basis of much of our mechanistic understanding have not yet fully explored the differences between TGF-β1, 2, and 3, there is evidence (like with ErbB ligands) for variation in affinity, timing, and so on, that likely drives biological diversity. These ligands, much like the EGF-like peptides, likely have overlapping but not identical GI functions within the superfamily. Additional detailed studies are needed to understand the functional differences between these molecules.
The receptors for TGF-β superfamily ligands have been shown to work in a coordinated manner, essentially in heterodimers of two classes of peptides. Ligand binding to TβRI results in activation of TβRII, leading to intracellular SMAD signaling, although SMAD-independent signaling as a result of TGF-β has also been shown. Receptor expression in the GI tract largely mirrors that of the ligands, with some variance in studies, largely supporting the thought that TGF-β acts along an autocrine or paracrine feedback loop.
A number of studies have demonstrated that signaling through TGF-β in the GI epithelium results in inhibition of cell growth through SMAD, whereas p38 signaling can elicit apoptosis and epithelial-to-mesenchymal transition. Growth inhibition by TGF-β involves cell cycle arrest in G1 phase and prevention of S-phase entry. Furthermore, in the GI tract, TGF-β can induce epithelial cell apoptosis and has been shown to function as a tumor suppressor. At first glance, this may seem at odds with the ligand’s original identification as a growth factor for transformed cells. In this regard, it is important to note that different studies support the role of TGF-β as either a tumor suppressor or a tumor promoter depending on context in the GI tract. In some cases, increased expression is seen as a marker of colorectal cancer severity and recurrence, and multiple colon carcinoma lines exhibit growth in response to stimulation with TGF-β. These altered outcomes are likely dependent on the specific genetic and microenvironment detail of a given tumor. TGF-β/SMAD signaling can also play important roles in regulation of the intestinal stem cell niche.
Under pathophysiological conditions, TGF-β stimulates wound healing at concentrations lower than required for growth arrest, and expression is upregulated in wound healing. Expression is increased by acute epithelial injury in the GI mucosa in patients with active inflammatory bowel diseases, suggesting this is a compensatory response to resolve disease. However, in contrast and making this observation more complex, impaired TGF-β signaling has been observed in some active IBD patients. In vivo studies show that the loss of TGF-β signaling renders mice more susceptible to chemically induced colitis.
Our understanding of the function of TGF-β has been greatly advanced through the use of genetic inactivation mouse models. TGF-β1 knockout mice that do not die in embryonic stages display aggressive inflammatory cell infiltration in the stomach and intestine, and tissue necrosis, which was an early indicator that TGF-β played important roles in tissue maintenance and had novel actions on inflammatory cells and immune homeostasis. These mice typically die by 20 days of age. Interestingly, this inflammation was not prevented by housing in germ-free environments, suggesting the microbiota is not precipitating this immune response. TGF-β2 and TGF-β3 knockout mice do not have obvious abnormalities in GI development, reinforcing the notion that TGF-β1 is the primary driver of GI outcomes.
The profound impact of TGF-β isoform knockout on inflammation is likely directly due to its expression and role within cells of the immune system. Macrophages, T-cells, and B-cells all express TGF-β, which can regulate pro- and antiinflammatory functions of these cells. The elevated inflammation in TGF-β1 KO mice points to an antiinflammatory role for TGF-β in the stomach and intestine. This is in line with the finding that TGF-β has an inhibitory effect on T-cell inflammatory activities. However, in vitro, TGF-β stimulates neutrophil chemotaxis, and is known to have both stimulatory and inhibitory effects on B-cell IgA production depending on context. In human intestinal mast cells, TGF-β tightly inhibits and regulates their function. Together, these findings demonstrate that, in addition to regulating epithelial cell behavior, TGF-β family ligands exert effects on multiple recruited immune cell types.
3.3.3
Other TGF-β Family Ligands
Bone morphogenetic protein (BMP) was discovered in 1965 as a bone remodeling factor. The family of TGF-β-related BMPs is extensive, including 6 of the originally identified BMPs (BMP2-7 but not BMP1, which is a metalloproteinase) as well as a number of more recently identified peptides totaling more than 15 family members. These growth factors can act through the same SMAD-dependent mechanism as canonical TGF-β signaling, and have proven to be essential for development of the GI tract during embryogenesis. BMP signaling is activated in all levels of gut patterning at the mesoderm, ectoderm, and endoderm layers of the developing chick embryo and in murine gut development. Temporally regulated BMP signaling is necessary for gastric patterning. Furthermore, this family is implicated in the regulation of intestinal stem cell self-renewal, as demonstrated by (among other results) expansion of the stem cell niche in conditional Bmpr1a null mice and the requirement for the BMP inhibitor Noggin in maintenance of Lgr5 + cell-dependent enteroid cultures. Thus, this signaling pathway is likely important in repair mechanisms of the GI tract. BMP7 administration ameliorated experimental colitis in rats, although in contrast BMP inhibitors have been shown to improve inflammation and colitis-associated anemia in mice. Dysregulated BMP activity has also been associated with development of Barretťs esophagus and eosinophilic esophagitis. BMPs can inhibit colon cancer cell growth or induce apoptosis in vitro, and loss of BMPs has been reported coincident with adenoma-cancer transition in colorectal cancer patients. On the other hand, at least some evidence also suggests a gain of function role in promoting tumor progression. The mechanisms driving these seemingly contradictory responses (balance of different BMP ligands, receptor expression, permissive microenvironment, etc.) require more investigation, but like other growth factors, the response and involvement of BMPs in intestinal disease are likely complex and context dependent.
Activins and inhibins are closely related ligands, generated by joining (through disulfide bonds) different subunits in five different combinations (Activin A, Activin B, Activin AB, Inhibin A, and Inhibin B). They are expressed in a variety of different patterns in either the mesenchyme or epithelium throughout the GI tract. They exert opposing effects on mitogenesis and other cellular functions, despite sharing similar signaling through the SMAD pathway. Furthermore, individual activins/inhibins have region-specific effects; for example, Activin A inhibits growth of gastric epithelium while stimulating colonic epithelial growth. Some evidence suggests that activins are regulated in IBD with expression of, for example, the βA subunit correlating with degree of inflammation in patients and mouse models. There is also some evidence linking altered Activin A expression to colon cancer, though like many TGF-β superfamily ligands the question of whether it is a promoter or inhibitor or tumor growth is likely context dependent. Since activins and inhibins regulate the immune system as well as the epithelium, further study will be required to understand where they exert their key effects during GI homeostasis and disease.
3.4
Insulin-Like Growth Factor Family
3.4.1
Introduction
The IGF ligands, including IGF-1 and IGF-2, were initially identified in 1957 as factors that increased sulfanation into rat cartilage, though not as the names they are known by today. Later studies in the 1970s independently identified them as molecules present in human serum and with a similar structure to proinsulin. Subsequent work revealed that IGFs have a substantial impact on cell metabolism growth and cell survival in the digestive system.
3.4.2
Insulin-Like Growth Factor (IGF)
Unlike insulin, IGFs are not produced and stored exclusively in the β cells of the pancreas, but are synthesized and secreted by a number of cells in the body in a regulated manner. IGF-1 is produced in adults by the mesenchyme of the intestine, and potentially other regions, and is known to stimulate intestinal epithelial cell proliferation. Both IGF-1 and IGF-2 are secreted in the breast milk as another route of reaching the GI tract. IGF-2 is commonly considered the embryonic form of IGF, with substantially higher expression during development, and is associated in adults with pathologic states such as cancer.
Largely these factors exert their effects on the GI tract by stimulating epithelial cell proliferation and inhibiting apoptosis. They act through endocrine, paracrine, and autocrine routes, binding and activating the IGFR-1 and IGFR-2 receptor tyrosine kinases ( Fig. 3.5 ). IGF-1 binds with high affinity to IGFR-1 and significantly lower affinity to IGFR-2. IGF-2 binds both IGFR-1 and IGFR-2 with high affinity. The majority of GI effects of IGFs are mediated by IGFR-1. Ligation of an IGFR leads to autophosphorylation of the receptor and activation of intracellular pathways, including insulin receptor substrate-1 (IRS-1). IRS-1 subsequently activates a variety of pathways, especially Ras and PI3K. Additionally, IGFR-1 can stimulate the heterotrimeric G-protein, Gi2, which alters signaling through ERK1/2 MAPK.

Regulation of IGF signaling is accomplished through several mechanisms. IGF-binding proteins (IGFBP) 1-6 can either promote or inhibit IGF function depending on cellular and environmental context, and also serve as transport proteins for IGFs throughout the body. Furthermore, in the intestine, IGFBPs are expressed in the lamina propria, implying a role in epithelial-mesenchymal interactions. An additional means of control is through compartmentalization of receptors in the GI epithelium. Similar to EGFR, IGFRs are primarily localized on the basolateral compartment of these cells, in vivo and in vitro.
The understanding of IGFs as significant regulators of cellular growth has in large part advanced through the use of in vivo models. In both IGFR-1 and IGF-1-deficient mice, animals are born significantly smaller than controls, grow poorly, and fail to thrive into adulthood. Secondary control of IGF signaling in the GI tract is highlighted by the finding that mice overexpressing IGF-1 exhibit normal GI epithelium and lamina propria, though smooth muscle throughout the body is hyperplastic and hypertrophic. On the other hand, rats receiving continuous administration of IGF-1 have significant growth of all GI organs and increased crypt and villus cell numbers. Mice heterozygous for IGF-1 have normal GI development, but fibrosis in the TNBS model of colitis was attenuated in this line. Oral delivery of porcine IGF-1-expressing Lactococcus lactis also attenuated DSS colitis in mice, suggesting potentially variable effects depending on localization of the ligand.
Evidence for proliferative and antiapoptotic effects of IGF-1 on the GI tract has been provided by studies in multiple experimental models. Oral feeding of IGF-1 to neonatal pigs increases small intestinal weight and small intestinal villus height. In mice that overexpress IGF-1 under control of the metallothionein-I promoter, small intestinal apoptosis was reduced both at baseline and in response to irradiation. Furthermore, these mice had greater small intestinal length and weight and increased villus height and crypt depth. Interestingly, endogenous growth hormone and IGF-1 gene expression were suppressed in these animals, suggesting the presence of a sensitive negative feedback loop. A wealth of other studies have been performed, reinforcing these findings (e.g., see Refs. ).
The role of IGF signaling has also been studied extensively in the context of disease. IGF-1 augments adaptive mucosal proliferation in rats that have undergone partial small intestinal resection and improves intestinal transplant outcomes in a rat model. The mucosal atrophy observed in the jejunum of parenterally fed rats is blunted by including IGF-1 in the formula, while IGF inhibitor attenuates intestinal adaptation following small bowel resection in zebrafish. Similarly, the small intestinal atrophy that occurs in chronic liver disease or sepsis is reduced by IGF-1. In cancer, a role for IGF was postulated due to observation that acromegaly, a disorder of dysregulated IGF and GH signaling, presented at elevated rates with colon cancer. Indeed, studies showed IGF-1, IGF-2, and IGF-1R to be increased in colorectal cancer. Findings suggest that they may be involved in the transformation of normal colon cells, and are implicated in chemotherapeutic drug resistance development. Serum levels of free IGF-1 are decreased while serum IGFBP3 is increased in type 1 diabetes, potentially contributing to intestinal inflammation and dysfunction in diabetic patients. In Crohn’s disease, IGF has also been implicated in the generation of fibrotic tissue, and reduced levels of IGF may contribute to growth failure observed with this disease. In line with this finding, some studies show decreased IGF-1 expression in IBD. These reports all provide evidence that proper IGF signaling is required for health, and dysregulation of this growth factor can contribute to disease.
3.5
Hepatocyte Growth Factor (HGF)
3.5.1
Introduction
Since its identification in the 1980s as factor driving hepatocyte proliferation/liver regeneration, HGF expression has been described throughout the body. It has many functional roles in human physiology, including significant roles in the development and repair of the fetal and adult digestive system. It signals with its cosignaling amplifier, HGF activator (HGFA), and through its receptor, the c-Met receptor tyrosine kinase, to elicit cell proliferation, motility, and differentiation ( Fig. 3.6 ). HGF signaling is also an important component of mucosal repair. Dysregulated HGF activity has been shown to be a feature of a number of diseases, including GI cancer.

3.5.2
Hepatocyte Growth Factor (HGF)
In the GI tract, HGF is predominantly synthesized by mesenchymal tissue as a proprotein (proHGF). Plasma-derived HGF secreted from the liver is also a source of this ligand in the adult gut, and milk-derived HGF is a source for the fetal GI tract. The precise regulation and control of HGF signaling are maintained in large part through activity of its cosignaling regulator, HGFA, a serine protease that converts proHGF to active ligand. Like HGF, HGFA is produced and secreted from the liver; it is also expressed at high levels in the GI tract in epithelial compartments that also express the HGF receptor, c-Met. Additionally, c-Met is primarily expressed basolaterally, which would place it in close association with its mesenchymally sourced ligand. A further control on HGF signaling is the expression of HGFA inhibitors types 1 and 2 (HAI-1 and HAI-2) and the expression of c-Met coreceptors such as CD44. The pattern of HGFA and HAI expression represents a mechanism of tight regulation on the activity of a widely produced ligand. Upon binding HGF, c-Met induces its cellular effects through multiple pathways, including JAK-STAT, Ras-MAPK, PI3K, and NF-κB.
HGF expression is induced by EGF, whereas TGF-β downregulates it. Its receptor, c-Met, is regulated by various factors including HGF itself, EGF, IL-1, IL-6, TNF, and several steroid hormones. The result of HGF signaling is increased growth of intestinal epithelial cells and enhanced wound healing/mucosal repair. HGF is also required for proper organ development, as it supports the generation of the enteric nervous system and the liver.
HGF signaling is a classic example of epithelial-mesenchymal communication and paracrine signaling of a growth factor in the GI tract. In the developing gut, it is a potent stimulator of growth in epithelial subpopulations. Furthermore, subepithelial intestinal fibroblasts potently stimulate the proliferation of epithelial cells via HGF release. The expression of the HGF coreceptor CD44 facilitates cell proliferation by direct activation of MEK/Erk signaling. Notably, in the stomach, CD44 is also required for epithelial progenitor proliferation. During development, HGF and c-Met are expressed by 6 weeks throughout the GI tract, and HGF-null mice are embryonic lethal. Conversely, mice overexpressing HGF develop tumors and other developmental abnormalities. In mice that specifically overexpress HGF in the GI tract, ulcerative proctitis, rectal prolapse, and intestinal pseudo-obstruction are observed.
In response to tissue injury, HGF and c-Met expressions are dramatically induced, and HAI-1 and CD44 are elevated in the regenerating epithelium. Induction is also evident in intestinal inflammatory diseases, though this is likely a protective response to injury as administering exogenous ligand ameliorates animal IBD models. Splice variant-specific isoforms of CD44 likely mediate some of these responses including epithelial regeneration. In response to epithelial injury, inflammatory cytokines like IL-1β appear to promote the release of HGFA/HAI-1 complexes from the cell surface, suggesting that HAI-1 may function as a reservoir of active HGFA in regions of epithelial repair, thus providing efficient localization to sites of mucosal injury. Interestingly, this repair appears to be dependent upon TGFβ signaling and may be involved in regulating the tight junctions between epithelial cells by altering the stability of E-cadherin and decreasing protein expression of E- and P-cadherins.
HGF signaling has been implicated in tumorigenesis and a wide range of tumor functions including cell motility, proliferation, invasion, progression, and neovascularization. HGF and c-Met are highly overexpressed in esophageal, stomach, colon, and rectal tumors. Furthermore, in some instances, c-Met activation can also be coopted and activated through interaction with other molecules such as the bacterially derived product cagA. The coreceptor CD44 has also been implicated in the development of gastric cancer. As HGF/c-Met signaling is increased on a variety of cancers and directly implicated in regulating cellular proliferation, a dysregulated feature of tumor cells, HGF signaling represents a promising target of oncogenic therapy.
3.6
Fibroblast Growth Factors
3.6.1
Introduction
FGF family is another growth factor family known to play an important role in the development, homeostasis, and injury/repair of the GI tract. It represents a family of 22 structurally similar peptides, divided into three groups based on their mode of action: canonical FGFs, intracellular FGFs, and endocrine or hormone-like FGFs. Each FGF ligand binds to one or more of the four described FGF receptors with tyrosine kinase activity: FGFR1, FGFR2, FGFR3, and FGFR4. Two splice variants (IIIb and IIIc forms) of FGFR1, FGFR2, and FGFR3 determine the specificity of their ligands. FGFR4, on the other hand, has no known splice variants.
3.6.2
Endocrine FGFs
Endocrine FGFs consist of 3 FGFs: FGF21, FGF23, and FGF15/19 (FGF15 is the mouse ortholog of human FGF19). Endocrine FGFs have very low affinity for FGFRs. Thus, the dimerization of FGFRs to klotho receptors (α and β) is necessary for the stabilization of ligand binding. FGF15 is the main endocrine FGF produced by the enterocytes, and is released in response to bile acid absorption in the ileum. Signaling of FGF15 to FGFR4 and β-klotho controls bile acid secretion and absorption; mice lacking FGF15, FGFR4, or β-klotho manifest increased synthesis of bile acids. On the other hand, FGF21 signaling is mainly active during the neonatal period, as FGF21 is present in breast milk and signals to abundant β-klotho in the lumen of the intestine. This interaction results in the secretion of digestive enzymes and intestinal hormones allowing for better lactose digestion and absorption. Lastly, FGF23, which mainly binds to FGFR1 and the coreceptor α-klotho, regulates phosphate absorption in the intestine through an unknown mechanism. Thus, endocrine FGF signaling in the intestine is necessary to maintain adequate digestive, absorptive, and endocrine functions ( Fig. 3.7 ).

3.6.3
Canonical FGFs
Canonical FGFs are the most extensively studied FGFs in the intestine. Several are present in the mouse intestine including FGF1, FGF2, FGF7, FGF9, and FGF10, whereas FGF3, FGF20, and FGF22 are absent. All four FGFRs are present in the intestine. FGFR1 and FGFR2 are expressed throughout the mucosa, while FGFR3 expression is confined to the lower half of the crypts, and FGFR4 is only expressed in the epithelium of the embryonic gut.
FGF1, also known as acidic FGF, is present in intestinal enterochromaffin cells and the myenteric plexus of the enteric nervous system in the colon. FGF1 administered in vivo prior to radiation injury increases crypt number, epithelial cell proliferation in the intestine, and crypt survival. In contrast, treatment with FGF1 after radiation injury did not have any rescue effect on the intestine. However, FGF1 displays a protective and antiapoptotic effect in response to dexamethasone-induced apoptosis in mouse intestine. A mutant form of FGF1, lacking its mitogenic activity, enhanced this antiapoptotic and protective effect, through maintaining ERK1/2 activation. FGF1 binds to all FGFRs, and could mediate its activity in the intestine through any of these receptors. However, given the special localization of FGFR3 isoform IIIb, predominantly present in the crypts of the small intestine and the colon, it is likely that FGF1 also plays an important role in the regulation of intestinal crypt and the fate determination of epithelial stem cells. Further studies are needed to investigate these possibilities. Similar to FGF1, FGF2 (also known as basic fibroblast growth factor) promotes intestinal growth and intestinal epithelial cell proliferation and differentiation. It is present in the mesenchyme of the intestine, but can also be secreted by regulatory T cells; it binds to FGFR1. FGF1 and FGF2 promote intestinal epithelial restitution by regulating TGF-β signaling. Furthermore, FGF2 is commonly used for the maintenance of stem cell pluripotency in vitro. Following radiation injury, FGF2 enhances the survival of intestinal stem cells in the crypt. Deletion of Fgf2 in mice protects the intestinal barrier from DSS-induced damage and cell death. FGF1 and FGF2 are both protective against ischemia reperfusion injury in rats via the regulation of P53 and ERK/P38 MAPK respectively. They also reduce intestinal inflammation in colitis as well as in ischemia/reperfusion injuries.
FGF4 is required in early development for hindgut specification. It acts in synergy with Wnt3a to induce the differentiation of pluripotent stem cells to ultimately give rise to the epithelial and mesenchymal intestinal cell types except for the enteric nervous system. Following radiation injury, it acts like FGF1 and FGF2, by promoting crypt cell survival and preventing apoptosis.
Administration of exogenous FGF7 (also known as keratinocyte growth factor, KGF) increases villus height, crypt depth, cell proliferation, and goblet cell differentiation in adult rat intestine. This increase in goblet cell differentiation is due to the regulation of intestinal trefoil factor/TFF3 by the goblet cell specific transcription factor GCSI-binding protein. In a disease context, FGF7 is secreted by fibroblasts or intraepithelial lymphocytes and acts on the epithelium by binding to FGFR1. Several studies have shown protective effects for FGF7 in colitis. In rat and mouse models of experimental colitis, administration of FGF7 prior to (but not after) the induction of colitis resulted in decreased ulcerations and cell death in the intestine. Moereover, FGF7 null mice present more severe damage in response to DSS as compared to wild-type animals. FGF7 also plays an important role in other intestinal diseases including short bowel syndrome and ischemia/reperfusion. Expression of both FGF7 and its receptor FGFR1 is significantly increased following massive short bowel resection in mice. FGF7 administration to rats that underwent small bowel resection results in increased crypt depth, villus height, and goblet cell differentiation. FGF7 also reduces cell death and dysregulation of tight junctions in ischemia reperfusion injury by upregulating IL-7, which in turn controls intraepithelial lymphocytes. This effect of FGF7 on IL-7 upregulation is abrogated by blocking FGFR2, thus suggesting that FGF7 signals via FGFR2 to induce upregulation of IL-7. In addition, FGF7 enhances epithelial healing in colonic anastomoses, where it increases cell proliferation and reduces inflammation. A truncated form of recombinant FGF7, called Palifermin or Kepivance, is currently clinically in use for the treatment of oral mucositis. However, despite the proven protective role of FGF7 in animal models of colitis, the administration of a growth factor with mitogen activity to treat patients with IBD is still under debate, as the risk of developing cancer raises questions regarding the long-term benefit of the drug.
FGF10 is another member of the family that appears to be important in intestinal development and disease. During development, lack of FGF10 results in duodenal, cecal, and colonic atresia, as well as anorectal malformations. This ligand is mostly present in the duodenum, cecum, and colon in mice. We recently demonstrated that it is present in the human ileum, and that overexpression of FGF10 in adult mice results in increased villus height, crypt depth, crypt cell proliferation, and goblet cell differentiation, and decreased Paneth cell numbers in the small intestine. Furthermore, in an in vitro model of mouse intestinal enteroids, exogenous FGF10 treatment resulted in the decrease of several stem cell markers (Lgr5, Lrig1, Hopx, Ascl2, and Sox9) and an increase in the intermediate secretory progenitor cells coexpressing Mmp7 and Muc2. Thus, FGF10 signaling plays an important role in intestinal development, lineage specification, and homeostasis. Additionally, FGF10 is a key growth factor in several intestinal diseases, such as IBD and short bowel syndrome. FGF10 expression is induced in the intestinal crypts following acute small bowel resection in mice, suggesting a role in the adaptation/repair process. It reduces disease severity, cell death, weight loss, and inflammatory cytokine secretion (IL-6, TNF, and IL-8) in mouse and rat models of DSS-induced colitis and indomethacin-induced ulceration. However, it should be noted that repifermin, a truncated form of FGF10, has been tested on 88 patients with active ulcerative colitis in a randomized clinical trial, and did not prove efficacious at reducing disease. Thus, further study is required to understand the role of this ligand in preventing or treating colitis and define the similarities and differences in this pathway in mice versus humans.
FGF9 is expressed in the mesenchyme and epithelium of the developing cecum and intestine, and is important for cecal formation and intestinal elongation during development. Global or mesenchymal specific deletion of FGF9 results in a severely hypoplastic cecum and shortening of the small intestine starting embryonic day E14.5. Lack of FGF9 results in decreased mesenchymal cell proliferation and premature differentiation of intestinal fibroblasts into myofibroblasts. Simultaneous deletion of mesenchymal FGFR1 and FGFR2 in mice results in similar intestinal phenotype obtained with FGF9 deletion. FGF9 binds to FGFR1, FGFR2, and FGFR3. FGF18 also binds to FGFR3. FGFR3 null mice have only half the number of stem cells and decreased Paneth cell number and increased crypt depth compared to wild-type mice. In contrast, the administration of FGF18 to adult mice results in decreased crypt cell proliferation. Increased levels of FGF9 and FGF18 are found in a mouse model of doxorubicin- induced damage, along with an expansion of Paneth cells, suggesting a role for these growth factors in Paneth cell differentiation. In vitro expression of FGFR3IIIb decreases in Caco-2 cells as they become more differentiated, but increases in response to an inflammatory stimulus such as IL-2 treatment. The exact role of FGFR3 and its ligands FGF9 and FGF18 in intestinal diseases is still poorly understood. Further studies are needed to elucidate the role and mechanisms through which these two growth factors act on intestinal stem cells and Paneth cell differentiation.
3.6.4
FGFs in GI Cancers
Like many other mitogenic factors, FGF ligands and their receptors are prominent players in carcinogenesis. Importantly, FGFs are targets of canonical Wnt signaling, a central pathway in cancer development and progression. The involvement of FGFs/FGFRs in different cancers has been reviewed in detail by Tanner and Grose in 2016. The FGF7/FGFR2 signaling axis is increased in diffuse gastric cancers. Furthermore, cancer-associated fibroblasts in the colon are known to secrete higher levels of FGF1 and FGF3 than normal fibroblasts. Conversely, neutralizing FGF1 and FGF3 or inhibiting their receptor FGFR4 reduces cancer cell proliferation and neovascularization in the colon, through the regulation of MMP7 and Erk. FGF20 is upregulated in human colon cancers, while it is nearly undetectable in normal colon tissues. Finally, FGF18 induces growth and survival of tumor cells in colorectal cancer and its expression is increased in those cancers.
3.7
Other Growth Factors in the Gastrointestinal Tract
Several other classes of growth factors have been identified over the past few decades that have mitogenic roles in the development and adult function of the GI tract, which we will touch on only briefly as they are discussed in depth in other chapters or in comprehensive reviews. These include trefoil family factors (TFF), the Wingless (Wnt) family, and Hedgehog (Hh) family proteins. These families each have distinct roles in regulating the physiology of the GI tract from embryonic to adult stags.
TFFs are expressed throughout the GI tract with regional specificity for individual molecules in the adult. These peptides—small, stable proteins produced by mucin-secreting epithelial cells —are critical for maintaining epithelial integrity and are involved in mucosal defense, tissue restitution, development, and inflammatory processes. They are secreted into the mucus barrier, where they maintain their structural and biological activities. Three distinct trefoil peptides have been identified in mammals: TFF1 (originally designated pS2), TFF2 (or spasmolytic polypeptide), and TFF3 (or intestinal trefoil factor). TFF1 and TFF2 expression is spatially restricted to gastric tissue with limited expression in the intestine (Brunner’s glands of the duodenum), whereas TFF3 is most highly expressed in intestinal tissue. Regulated expression of these proteins is known to be controlled by various factors. For instance, TFF1 is responsive to insulin, IGF-1, FGF, and retinoic acid, TFF2 is silenced in response to Helicobacter pylori infection in the stomach, and TFF3 responds to neuropeptides, acetylcholine, hypoxia, and short-chain fatty acids. Notably, TFF3 levels appear to be linked to expression of Muc2, an important glycoprotein critical to limiting inflammation in the intestinal mucosa, through sharing a goblet cell response element for transcriptional control. It has been hypothesized that one aspect of TFF function is through cross-linking with mucin glycoproteins to reinforce the mucus barrier. Through this mechanism and the ability to regulate packaging and secretion of mucin glycoproteins, it is evident TFFs play a key role in establishing and maintaining the mucosal epithelial surface, with coordinated biological activities aimed at promoting GI homeostasis. A number of recent and thorough reviews are available on the roles and functions of trefoil factors.
The diverse family of secreted Wnt ligands has significant impact on embryonic development of the GI tract. Recent studies have also implicated them in GI tissue renewal and maintenance. In particular, Wnt signaling is believed to be a principal force regulating GI regional specification. Wnts signal through the Frizzled family of cell surface receptors and LRP receptors that implement β-catenin and Ca 2 + signaling outcomes. A preponderance of ligands and receptors reflects the diversity of signaling outcomes observed within this family. The importance of Wnt signaling in maintaining the GI tract has been highlighted in the past several years by lineage tracing experiments in mice, and by the development of the GI organoid culture system. These 3D epithelial cultures rely on recombinant Wnt ligand for the establishment and expansion of epithelial cultures generated from either isolated stem cells or isolated crypts. Interestingly, removal of Wnt signaling promotes epithelial differentiation, indicating Wnt and β-catenin promotion are important signals in the self-renewal of the GI stem cell niche. A detailed description of Wnt signaling in the GI tract is provided in Chapter 9 .
Hedgehog (Hh) ligands and their receptors each play roles in not only the development of the GI tract but also in the maintenance of adult GI mucosa. Humans express three Hh proteins: Sonic Hedgehog (Shh), Indian Hedgehog (Ihh), and Desert Hedgehog (Dhh). Shh and Ihh are necessary for appropriate regional development of the gut. These ligands signal via the Glioma family of oncogenic transcription factors (Gli1-3). In the adult, Shh is predominantly involved in maintenance of the gastric epithelium and differentiation, whereas Ihh is involved in the homeostasis of the intestinal tract. Hh ligands are also secreted into circulation. Shh in particular is expressed in and secreted primarily from gastric parietal cells. This ligand is a biologically active signaling molecule with long- and short-range action. In the pancreas, members of the Hh pathway are overexpressed in cancer, and elevated Hh correlates with progression to metastasis. Furthermore, Hh signaling is elevated in liver responses to disease suggesting dysregulation of this pathway is a feature or outcome of liver injury. These findings show a significant role for Hh in homeostasis and disease. The role of Hh signaling in the GI tract is described in detail in Chapter 10 .
3.8
Conclusion
The discovery and study of growth factors over the past seven decades has revealed a complex interplay of regulatory mechanisms that exquisitely control location, timing, and strength of mitogenic signaling in the body. Defining these mechanisms has been foundational for understanding how the GI tract develops, functions, defends, repairs, and maintains itself. Furthermore, the study of growth factors has revealed the many pathways and redundancies that exist for maintenance and function of GI tissues.
Understanding the basic physiological processes by which growth factors operate and signal has been instrumental to identifying specific roles in health and disease, sometimes decades after the initial findings were made. These findings have led to advanced research and clinical applications (e.g., receptor tyrosine kinase inhibitors, metalloproteinase inhibitors, TGF-β inhibitors). Therapeutics that elicit growth factor signaling, or antagonists to these pathways, is being realized in the clinic today. Use of growth factors or additional avenues of targeting growth factor activity will likely be therapeutically useful in the near future in GI diseases such as inflammatory bowel disease, necrotizing enterocolitis, and GI cancers. Furthermore, as our tools progress for understanding the development and regeneration of GI tissues, our understanding of the impact that growth factors have in these areas will continue to expand and be refined, enabling precise control of the beneficial aspects of growth factor signaling while restraining the deleterious effects.
References
- 1. Naresh A., et al: The ERBB4/HER4 intracellular domain 4ICD is a BH3-only protein promoting apoptosis of breast cancer cells. Cancer Res 2006; 66: pp. 6412-6420
- 2. Schumacher M.A., et al: ErbB4 signaling stimulates pro-inflammatory macrophage apoptosis and limits colonic inflammation. Cell Death Dis 2017; 8: pp. e2622
- 3. Keely S.J., Uribe J.M., and Barrett K.E.: Carbachol stimulates transactivation of epidermal growth factor receptor and mitogen-activated protein kinase in T84 cells. Implications for carbachol-stimulated chloride secretion. J Biol Chem 1998; 273: pp. 27111-27117
- 4. McCole D.F., et al: Epidermal growth factor partially restores colonic ion transport responses in mouse models of chronic colitis. Gastroenterology 2005; 129: pp. 591-608
- 5. Ciacci C., Lind S.E., and Podolsky D.K.: Transforming growth factor beta regulation of migration in wounded rat intestinal epithelial monolayers. Gastroenterology 1993; 105: pp. 93-101
- 6. Kurokowa M., Lynch K., and Podolsky D.K.: Effects of growth factors on an intestinal epithelial cell line: transforming growth factor beta inhibits proliferation and stimulates differentiation. Biochem Biophys Res Commun 1987; 142: pp. 775-782
- 7. Tao Q., et al: Vitamin D prevents the intestinal fibrosis via induction of vitamin D receptor and inhibition of transforming growth factor-beta1/Smad3 pathway. Dig Dis Sci 2015; 60: pp. 868-875
- 8. Loeuillard E., et al: 2,4,6-Trinitrobenzene sulfonic acid-induced chronic colitis with fibrosis and modulation of TGF-beta1 signaling. World J Gastroenterol 2014; 20: pp. 18207-18215
- 9. Li C., and Kuemmerle J.F.: Mechanisms that mediate the development of fibrosis in patients with Crohn’s disease. Inflamm Bowel Dis 2014; 20: pp. 1250-1258
- 10. Li C., et al: Noncanonical STAT3 activation regulates excess TGF-beta1 and collagen I expression in muscle of stricturing Crohn’s disease. J Immunol 2015; 194: pp. 3422-3431
- 11. Polyak K.: Negative regulation of cell growth by TGF beta. Biochim Biophys Acta 1996; 1242: pp. 185-199
- 12. Zhu Y., et al: Smad3 mutant mice develop metastatic colorectal cancer. Cell 1998; 94: pp. 703-714
- 13. Koyama S.Y., and Podolsky D.K.: Differential expression of transforming growth factors alpha and beta in rat intestinal epithelial cells. J Clin Invest 1989; 83: pp. 1768-1773
- 14. Kommoss F., et al: In situ distribution of transforming growth factor alpha in normal human tissues and in malignant tumours of the ovary. J Pathol 1990; 162: pp. 223-230
- 15. Koliaraki V., Roulis M., and Kollias G.: Tpl2 regulates intestinal myofibroblast HGF release to suppress colitis-associated tumorigenesis. J Clin Invest 2012; 122: pp. 4231-4242
- 16. Petrides P.E., et al: Isolation and characterization of epidermal growth factor from human milk. FEBS Lett 1985; 187: pp. 89-95
- 17. Moran J.R., et al: Epidermal growth factor in human milk: daily production and diurnal variation during early lactation in mothers delivering at term and at premature gestation. J Pediatr 1983; 103: pp. 402-405
- 18. Michalsky M.P., et al: Heparin-binding EGF-like growth factor is present in human amniotic fluid and breast milk. J Pediatr Surg 2002; 37: pp. 1-6
- 19. McElroy S.J., et al: The ErbB4 ligand neuregulin-4 protects against experimental necrotizing enterocolitis. Am J Pathol 2014; 184: pp. 2768-2778
- 20. Marti U., Burwen S.J., and Jones A.L.: Biological effects of epidermal growth factor, with emphasis on the gastrointestinal tract and liver: an update. Hepatology 1989; 9: pp. 126-138
- 21. Frey M.R., et al: The ErbB4 growth factor receptor is required for colon epithelial cell survival in the presence of TNF. Gastroenterology 2009; 136: pp. 217-226
- 22. Feng Y., and Teitelbaum D.H.: Epidermal growth factor/TNF-alpha transactivation modulates epithelial cell proliferation and apoptosis in a mouse model of parenteral nutrition. Am J Physiol Gastrointest Liver Physiol 2012; 302: pp. G236-G249
- 23. Barnard J.A., et al: Auto- and cross-induction within the mammalian epidermal growth factor-related peptide family. J Biol Chem 1994; 269: pp. 22817-22822
- 24. Cohen S.: Isolation of a mouse submaxillary gland protein accelerating incisor eruption and eyelid opening in the new-born animal. J Biol Chem 1962; 237: pp. 1555-1562
- 25. Singh B., Carpenter G., and Coffey R.J.: EGF receptor ligands: recent advances. F1000Res 2016; 5:
- 26. Roskoski R.: The ErbB/HER family of protein-tyrosine kinases and cancer. Pharmacol Res 2014; 79: pp. 34-74
- 27. Garrett T.P., et al: The crystal structure of a truncated ErbB2 ectodomain reveals an active conformation, poised to interact with other ErbB receptors. Mol Cell 2003; 11: pp. 495-505
- 28. Citri A., Skaria K.B., and Yarden Y.: The deaf and the dumb: the biology of ErbB-2 and ErbB-3. Exp Cell Res 2003; 284: pp. 54-65
- 29. Lin C.R., et al: Expression cloning of human EGF receptor complementary DNA: gene amplification and three related messenger RNA products in A431 cells. Science 1984; 224: pp. 843-848
- 30. Shi F., et al: ErbB3/HER3 intracellular domain is competent to bind ATP and catalyze autophosphorylation. Proc Natl Acad Sci U S A 2010; 107: pp. 7692-7697
- 31. Guy P.M., et al: Insect cell-expressed p180erbB3 possesses an impaired tyrosine kinase activity. Proc Natl Acad Sci U S A 1994; 91: pp. 8132-8136
- 32. Kaushansky A., et al: System-wide investigation of ErbB4 reveals 19 sites of Tyr phosphorylation that are unusually selective in their recruitment properties. Chem Biol 2008; 15: pp. 808-817
- 33. Jones J.T., Akita R.W., and Sliwkowski M.X.: Binding specificities and affinities of egf domains for ErbB receptors. FEBS Lett 1999; 447: pp. 227-231
- 34. Wang Z., et al: Endocytosis deficiency of epidermal growth factor (EGF) receptor-ErbB2 heterodimers in response to EGF stimulation. Mol Biol Cell 1999; 10: pp. 1621-1636
- 35. Shen F., et al: Identification of the domain in ErbB2 that restricts ligand-induced degradation. Cell Signal 2008; 20: pp. 779-786
- 36. Heitz P.U., et al: Immunohistochemical localisation of urogastrone to human duodenal and submandibular glands. Gut 1978; 19: pp. 408-413
- 37. Cohen S., and Carpenter G.: Human epidermal growth factor: isolation and chemical and biological properties. Proc Natl Acad Sci U S A 1975; 72: pp. 1317-1321
- 38. Playford R.J., et al: The epidermal growth factor receptor (EGF-R) is present on the basolateral, but not the apical, surface of enterocytes in the human gastrointestinal tract. Gut 1996; 39: pp. 262-266
- 39. Lu N., et al: Activation of the epidermal growth factor receptor in macrophages regulates cytokine production and experimental colitis. J Immunol 2014; 192: pp. 1013-1023
- 40. Hardbower D.M., et al: EGFR regulates macrophage activation and function in bacterial infection. J Clin Invest 2016; 126: pp. 3296-3312
- 41. Avissar N.E., et al: Epidermal growth factor receptor is increased in rabbit intestinal brush border membrane after small bowel resection. Dig Dis Sci 2000; 45: pp. 1145-1152
- 42. Miro L., et al: Aldosterone induces myofibroblast EGF secretion to regulate epithelial colonic permeability. Am J Physiol Cell Physiol 2013; 304: pp. C918-C926
- 43. Sato T., et al: Paneth cells constitute the niche for Lgr5 stem cells in intestinal crypts. Nature 2011; 469: pp. 415-418
- 44. Poulsen S.S., et al: Immunohistochemical localization of epidermal growth factor in rat and man. Histochemistry 1986; 85: pp. 389-394
- 45. Wright N.A., Pike C., and Elia G.: Induction of a novel epidermal growth factor-secreting cell lineage by mucosal ulceration in human gastrointestinal stem cells. Nature 1990; 343: pp. 82-85
- 46. Dvorak B.: Milk epidermal growth factor and gut protection. J Pediatr 2010; 156: pp. S31-S35
- 47. Kang P., et al: Epidermal growth factor-expressing . J Nutr 2010; 140: pp. 806-811
- 48. Bedford A., et al: Epidermal growth factor containing culture supernatant enhances intestine development of early-weaned pigs in vivo: potential mechanisms involved. J Biotechnol 2015; 196-197: pp. 9-19
- 49. Yamaoka T., et al: Specific epidermal growth factor receptor autophosphorylation sites promote mouse colon epithelial cell chemotaxis and restitution. Am J Physiol Gastrointest Liver Physiol 2011; 301: pp. G368-G376
- 50. Riegler M., et al: Epidermal growth factor promotes rapid response to epithelial injury in rabbit duodenum in vitro. Gastroenterology 1996; 111: pp. 28-36
- 51. Polk D.B.: Epidermal growth factor receptor-stimulated intestinal epithelial cell migration requires phospholipase C activity. Gastroenterology 1998; 114: pp. 493-502
- 52. Frey M.R., Golovin A., and Polk D.B.: Epidermal growth factor-stimulated intestinal epithelial cell migration requires Src family kinase-dependent p38 MAPK signaling. J Biol Chem 2004; 279: pp. 44513-44521
- 53. Goodlad R.A., et al: Proliferative effects of urogastrone-EGF on the intestinal epithelium. Gut 1987; 28(Suppl.): pp. 37-43
- 54. Al-Nafussi A.I., and Wright N.A.: The effect of epidermal growth factor (EGF) on cell proliferation of the gastrointestinal mucosa in rodents. Virchows Arch B Cell Pathol Incl Mol Pathol 1982; 40: pp. 63-69
- 55. Miguel J.C., et al: Epidermal growth factor suppresses intestinal epithelial cell shedding through a MAPK-dependent pathway. J Cell Sci 2017; 130: pp. 90-96
- 56. Wolfgang C.L., et al: Epidermal growth factor activation of intestinal glutamine transport is mediated by mitogen-activated protein kinases. J Gastrointest Surg 2003; 7: pp. 149-156
- 57. van der Merwe J.Q., Hollenberg M.D., and MacNaughton W.K.: EGF receptor transactivation and MAP kinase mediate proteinase-activated receptor-2-induced chloride secretion in intestinal epithelial cells. Am J Physiol Gastrointest Liver Physiol 2008; 294: pp. G441-G451
- 58. Paul G., et al: Interferon-gamma alters downstream signaling originating from epidermal growth factor receptor in intestinal epithelial cells: functional consequences for ion transport. J Biol Chem 2012; 287: pp. 2144-2155
- 59. Mroz M.S., and Keely S.J.: Epidermal growth factor chronically upregulates Ca(2 . J Physiol 2012; 590: pp. 1907-1920
- 60. Horvath K., et al: Short-term effect of epidermal growth factor (EGF) on sodium and glucose cotransport of isolated jejunal epithelial cells. Biochim Biophys Acta 1994; 1222: pp. 215-222
- 61. Berlanga-Acosta J., et al: Gastrointestinal cell proliferation and crypt fission are separate but complementary means of increasing tissue mass following infusion of epidermal growth factor in rats. Gut 2001; 48: pp. 803-807
- 62. Powell A.E., et al: The pan-ErbB negative regulator Lrig1 is an intestinal stem cell marker that functions as a tumor suppressor. Cell 2012; 149: pp. 146-158
- 63. Scopelliti A., et al: Local control of intestinal stem cell homeostasis by enteroendocrine cells in the adult Drosophila midgut. Curr Biol 2014; 24: pp. 1199-1211
- 64. Sato T., and Clevers H.: Growing self-organizing mini-guts from a single intestinal stem cell: mechanism and applications. Science 2013; 340: pp. 1190-1194
- 65. Schumacher M.A., et al: The use of murine-derived fundic organoids in studies of gastric physiology. J Physiol 2015; 593: pp. 1809-1827
- 66. Sorkin A., and Goh L.K.: Endocytosis and intracellular trafficking of ErbBs. Exp Cell Res 2009; 315: pp. 683-696
- 67. Wang L., et al: Berberine inhibits proliferation and down-regulates epidermal growth factor receptor through activation of Cbl in colon tumor cells. PLoS One 2013; 8: pp. e56666
- 68. Playford R.J., et al: Epidermal growth factor is digested to smaller, less active forms in acidic gastric juice. Gastroenterology 1995; 108: pp. 92-101
- 69. Bower J.M., et al: The inhibition of gastric acid secretion by epidermal growth factor. Experientia 1975; 31: pp. 825-826
- 70. Frey M.R., et al: p38 kinase regulates epidermal growth factor receptor downregulation and cellular migration. EMBO J 2006; 25: pp. 5683-5692
- 71. Hirano M., et al: Epidermal growth factor enhances repair of rat intestinal mucosa damaged by oral administration of methotrexate. J Gastroenterol 1995; 30: pp. 169-176
- 72. Raper S.E., et al: Translocation of epidermal growth factor to the hepatocyte nucleus during rat liver regeneration. Gastroenterology 1987; 92: pp. 1243-1250
- 73. Earp H.S., et al: Epidermal growth factor (EGF) and hormones stimulate phosphoinositide hydrolysis and increase EGF receptor protein synthesis and mRNA levels in rat liver epithelial cells. Evidence for protein kinase C-dependent and -independent pathways. J Biol Chem 1988; 263: pp. 13868-13874
- 74. Dvorak B., et al: Epidermal growth factor reduces the development of necrotizing enterocolitis in a neonatal rat model. Am J Physiol Gastrointest Liver Physiol 2002; 282: pp. G156-G164
- 75. Egger B., et al: Mice harboring a defective epidermal growth factor receptor (waved-2) have an increased susceptibility to acute dextran sulfate-induced colitis. Scand J Gastroenterol 2000; 35: pp. 1181-1187
- 76. Dube P.E., et al: Epidermal growth factor receptor inhibits colitis-associated cancer in mice. J Clin Invest 2012; 122: pp. 2780-2792
- 77. Egger B., et al: Reduced susceptibility of mice overexpressing transforming growth factor alpha to dextran sodium sulphate induced colitis. Gut 1998; 43: pp. 64-70
- 78. Sinha A., et al: Epidermal growth factor enemas with oral mesalamine for mild-to-moderate left-sided ulcerative colitis or proctitis. N Engl J Med 2003; 349: pp. 350-357
- 79. Clark J.A., et al: Intestinal barrier failure during experimental necrotizing enterocolitis: protective effect of EGF treatment. Am J Physiol Gastrointest Liver Physiol 2006; 291: pp. G938-G949
- 80. Good M., et al: Breast milk protects against the development of necrotizing enterocolitis through inhibition of Toll-like receptor 4 in the intestinal epithelium via activation of the epidermal growth factor receptor. Mucosal Immunol 2015; 8: pp. 1166-1179
- 81. Clark J.A., et al: Epidermal growth factor reduces intestinal apoptosis in an experimental model of necrotizing enterocolitis. Am J Physiol Gastrointest Liver Physiol 2005; 288: pp. G755-G762
- 82. Halpern M.D., et al: Bile acids induce ileal damage during experimental necrotizing enterocolitis. Gastroenterology 2006; 130: pp. 359-372
- 83. Sullivan P.B., et al: Intestinal mucosa remodeling by recombinant human epidermal growth factor(1-48) in neonates with severe necrotizing enterocolitis. J Pediatr Surg 2007; 42: pp. 462-469
- 84. Frey M.R., and Brent Polk D.: ErbB receptors and their growth factor ligands in pediatric intestinal inflammation. Pediatr Res 2014; 75: pp. 127-132
- 85. Punt C.J., Koopman M., and Vermeulen L.: From tumour heterogeneity to advances in precision treatment of colorectal cancer. Nat Rev Clin Oncol 2017; 14: pp. 235-246
- 86. Mendelsohn J., and Baselga J.: Epidermal growth factor receptor targeting in cancer. Semin Oncol 2006; 33: pp. 369-385
- 87. de Castro-Carpeno J., et al: EGFR and colon cancer: a clinical view. Clin Transl Oncol 2008; 10: pp. 6-13
- 88. Korc M., et al: Overexpression of the epidermal growth factor receptor in human pancreatic cancer is associated with concomitant increases in the levels of epidermal growth factor and transforming growth factor alpha. J Clin Invest 1992; 90: pp. 1352-1360
- 89. Barton C.M., et al: Transforming growth factor alpha and epidermal growth factor in human pancreatic cancer. J Pathol 1991; 163: pp. 111-116
- 90. Yoshida K., et al: EGF and TGF-alpha, the ligands of hyperproduced EGFR in human esophageal carcinoma cells, act as autocrine growth factors. Int J Cancer 1990; 45: pp. 131-135
- 91. Yoshida K., et al: Expression of epidermal growth factor, transforming growth factor-alpha and their receptor genes in human gastric carcinomas; implication for autocrine growth. Jpn J Cancer Res 1990; 81: pp. 43-51
- 92. Filipe M.I., et al: Expression of transforming growth factor alpha, epidermal growth factor receptor and epidermal growth factor in precursor lesions to gastric carcinoma. Br J Cancer 1995; 71: pp. 30-36
- 93. Ostrowski J., et al: Increased activity of MAP, p70S6 and p90rs kinases is associated with AP-1 activation in spontaneous liver tumours, but not in adjacent tissue in mice. Br J Cancer 2000; 82: pp. 1041-1050
- 94. Messa C., et al: EGF, TGF-alpha, and EGF-R in human colorectal adenocarcinoma. Acta Oncol 1998; 37: pp. 285-289
- 95. Borlak J., et al: Epidermal growth factor-induced hepatocellular carcinoma: gene expression profiles in precursor lesions, early stage and solitary tumours. Oncogene 2005; 24: pp. 1809-1819
- 96. Troyer K.L., et al: Growth retardation, duodenal lesions, and aberrant ileum architecture in triple null mice lacking EGF, amphiregulin, and TGF-alpha. Gastroenterology 2001; 121: pp. 68-78
- 97. Luetteke N.C., et al: Targeted inactivation of the EGF and amphiregulin genes reveals distinct roles for EGF receptor ligands in mouse mammary gland development. Development 1999; 126: pp. 2739-2750
- 98. Ronan T., et al: Different epidermal growth factor receptor (EGFR) agonists produce unique signatures for the recruitment of downstream signaling proteins. J Biol Chem 2016; 291: pp. 5528-5540
- 99. Miettinen P.J., et al: Epithelial immaturity and multiorgan failure in mice lacking epidermal growth factor receptor. Nature 1995; 376: pp. 337-341
- 100. Anzano M.A., et al: Synergistic interaction of two classes of transforming growth factors from murine sarcoma cells. Cancer Res 1982; 42: pp. 4776-4778
- 101. Massague J.: Type beta transforming growth factor from feline sarcoma virus-transformed rat cells. Isolation and biological properties. J Biol Chem 1984; 259: pp. 9756-9761
- 102. Perez-Tomas R., Cullere X., and Diaz C.: Immunohistochemical localization of transforming growth factor alpha in the developing rat colon. Gastroenterology 1993; 104: pp. 789-795
- 103. Malden L.T., Novak U., and Burgess A.W.: Expression of transforming growth factor alpha messenger RNA in the normal and neoplastic gastro-intestinal tract. Int J Cancer 1989; 43: pp. 380-384
- 104. Hormi K., and Lehy T.: Developmental expression of transforming growth factor-alpha and epidermal growth factor receptor proteins in the human pancreas and digestive tract. Cell Tissue Res 1994; 278: pp. 439-450
- 105. Miettinen P.J.: Transforming growth factor-alpha and epidermal growth factor expression in human fetal gastrointestinal tract. Pediatr Res 1993; 33: pp. 481-486
- 106. Kelly E.J., et al: Role of epidermal growth factor and transforming growth factor alpha in the developing stomach. Arch Dis Child Fetal Neonatal Ed 1997; 76: pp. F158-F162
- 107. Autenrieth I.B., et al: Cytokine mRNA expression in intestinal tissue of interleukin-2 deficient mice with bowel inflammation. Gut 1997; 41: pp. 793-800
- 108. Thomas D.M., et al: Immunoreactivity of transforming growth factor alpha in the normal adult gastrointestinal tract. Gut 1992; 33: pp. 628-631
- 109. Natale G., et al: Differential distribution of transforming growth factor-alpha immunohistochemistry within whole gastric mucosa in rats. Eur J Histochem 2003; 47: pp. 359-364
- 110. Nasim M.M., et al: Transforming growth factor alpha expression in normal gastric mucosa, intestinal metaplasia, dysplasia and gastric carcinoma—an immunohistochemical study. Histopathology 1992; 20: pp. 339-343
- 111. Cartlidge S.A., and Elder J.B.: Transforming growth factor alpha and epidermal growth factor levels in normal human gastrointestinal mucosa. Br J Cancer 1989; 60: pp. 657-660
- 112. Bluth R.F., et al: Immunolocalization of transforming growth factor-alpha in normal and diseased human gastric mucosa. Hum Pathol 1995; 26: pp. 1333-1340
- 113. Beauchamp R.D., et al: Localization of transforming growth factor alpha and its receptor in gastric mucosal cells. Implications for a regulatory role in acid secretion and mucosal renewal. J Clin Invest 1989; 84: pp. 1017-1023
- 114. Xian C.J., Mardell C.E., and Read L.C.: Specificity of the localization of transforming growth factor-alpha immunoreactivity in colon mucosa. J Histochem Cytochem 1999; 47: pp. 949-958
- 115. Fujiwara Y., et al: Increased expression of transforming growth factor- alpha and epidermal growth factor receptors in rat chronic reflux esophagitis. J Gastroenterol Hepatol 2004; 19: pp. 521-527
- 116. Cameron I.L., and Hardman W.E.: Paracrine action of transforming growth factor-alpha in rectal crypt epithelium of humans. Cell Biol Int 2002; 26: pp. 1029-1034
- 117. Calabro A., et al: Expression of epidermal growth factor, transforming growth factor-alpha and their receptor in the human oesophagus. Histochem J 1997; 29: pp. 745-758
- 118. Peschon J.J., et al: An essential role for ectodomain shedding in mammalian development. Science 1998; 282: pp. 1281-1284
- 119. Hinkle C.L., et al: Multiple metalloproteinases process protransforming growth factor-alpha (proTGF-alpha). Biochemistry 2003; 42: pp. 2127-2136
- 120. Kudlow J.E., and Bjorge J.D.: TGF alpha in normal physiology. Semin Cancer Biol 1990; 1: pp. 293-302
- 121. Dempsey P.J., et al: Apical enrichment of human EGF precursor in Madin-Darby canine kidney cells involves preferential basolateral ectodomain cleavage sensitive to a metalloprotease inhibitor. J Cell Biol 1997; 138: pp. 747-758
- 122. Dempsey P.J., and Coffey R.J.: Basolateral targeting and efficient consumption of transforming growth factor-alpha when expressed in Madin-Darby canine kidney cells. J Biol Chem 1994; 269: pp. 16878-16889
- 123. Winkler M.E., et al: Epidermal growth factor and transforming growth factor alpha bind differently to the epidermal growth factor receptor. Biochemistry 1989; 28: pp. 6373-6378
- 124. Lax I., et al: Chicken epidermal growth factor (EGF) receptor: cDNA cloning, expression in mouse cells, and differential binding of EGF and transforming growth factor alpha. Mol Cell Biol 1988; 8: pp. 1970-1978
- 125. Ebner R., and Derynck R.: Epidermal growth factor and transforming growth factor-alpha: differential intracellular routing and processing of ligand-receptor complexes. Cell Regul 1991; 2: pp. 599-612
- 126. Ouyang X., et al: Transforming growth factor-alpha short-circuits downregulation of the epidermal growth factor receptor. J Cell Physiol 1999; 179: pp. 52-57
- 127. Sizeland A.M., and Burgess A.W.: Anti-sense transforming growth factor alpha oligonucleotides inhibit autocrine stimulated proliferation of a colon carcinoma cell line. Mol Biol Cell 1992; 3: pp. 1235-1243
- 128. Rutten M.J., et al: TGF-alpha is a potent mitogen for primary cultures of guinea pig gastric mucous epithelial cells. Am J Physiol 1993; 265: pp. G361-G369
- 129. Chen M.C., Lee A.T., and Soll A.H.: Mitogenic response of canine fundic epithelial cells in short-term culture to transforming growth factor alpha and insulinlike growth factor I. J Clin Invest 1991; 87: pp. 1716-1723
- 130. Barnard J.A., et al: Epidermal growth factor-related peptides and their relevance to gastrointestinal pathophysiology. Gastroenterology 1995; 108: pp. 564-580
- 131. Acra S.A., et al: Increased intestinal epithelial proliferation in metallothioneine-transforming growth factor alpha transgenic mice. Regul Pept 1998; 74: pp. 105-112
- 132. Playford R.J., et al: Comparison of the effects of transforming growth factor alpha and epidermal growth factor on gastrointestinal proliferation and hormone release. Digestion 1996; 57: pp. 362-367
- 133. Sharp R., et al: Transforming growth factor alpha disrupts the normal program of cellular differentiation in the gastric mucosa of transgenic mice. Development 1995; 121: pp. 149-161
- 134. Goldenring J.R., et al: Overexpression of transforming growth factor- alpha alters differentiation of gastric cell lineages. Dig Dis Sci 1996; 41: pp. 773-784
- 135. Egger B., et al: Mice lacking transforming growth factor alpha have an increased susceptibility to dextran sulfate-induced colitis. Gastroenterology 1997; 113: pp. 825-832
- 136. Kokudo N., et al: Transforming growth factor-alpha (TGF-alpha) improves hepatic DNA synthesis after hepatectomy in cirrhotic rats. J Surg Res 1992; 52: pp. 648-655
- 137. Russell W.E., et al: Liver regeneration and hepatocarcinogenesis in transforming growth factor-alpha-targeted mice. Mol Carcinog 1996; 15: pp. 183-189
- 138. Sandgren E.P., et al: Overexpression of TGF alpha in transgenic mice: induction of epithelial hyperplasia, pancreatic metaplasia, and carcinoma of the breast. Cell 1990; 61: pp. 1121-1135
- 139. Jhappan C., et al: TGF alpha overexpression in transgenic mice induces liver neoplasia and abnormal development of the mammary gland and pancreas. Cell 1990; 61: pp. 1137-1146
- 140. Coffey R.J., et al: Roles for transforming growth factor-alpha in gastric physiology and pathophysiology. Yale J Biol Med 1992; 65: pp. 693-704
- 141. Uribe J.M., and Barrett K.E.: Nonmitogenic actions of growth factors: an integrated view of their role in intestinal physiology and pathophysiology. Gastroenterology 1997; 112: pp. 255-268
- 142. Yacoub L., Goldman H., and Odze R.D.: Transforming growth factor-alpha, epidermal growth factor receptor, and MiB-1 expression in Barretťs-associated neoplasia: correlation with prognosis. Mod Pathol 1997; 10: pp. 105-112
- 143. D’Errico A., et al: Role and new perspectives of transforming growth factor-alpha (TGF-alpha) in adenocarcinoma of the gastro-oesophageal junction. Br J Cancer 2000; 82: pp. 865-870
- 144. Yamamoto T., Hattori T., and Tahara E.: Interaction between transforming growth factor-alpha and c-Ha-ras p21 in progression of human gastric carcinoma. Pathol Res Pract 1988; 183: pp. 663-669
- 145. Suzuki T., et al: Growth of human gastric carcinomas and expression of epidermal growth factor, transforming growth factor-alpha, epidermal growth factor receptor and p185c-erbB-2. Oncology 1995; 52: pp. 385-391
- 146. Muller W., and Borchard F.: Expression of transforming growth factor-alpha in gastric carcinoma and normal gastric mucosa cells. Cancer 1992; 69: pp. 2871-2875
- 147. Choi J.H., et al: Detection of transforming growth factor-alpha in the serum of gastric carcinoma patients. Oncology 1999; 57: pp. 236-241
- 148. Tanaka S., et al: Immunoreactive transforming growth factor-alpha and epidermal growth factor in colorectal adenomas and carcinomas. Oncology 1992; 49: pp. 381-385
- 149. Shim K.S., et al: Increased serum levels of transforming growth factor-alpha in patients with colorectal cancer. Dis Colon Rectum 1998; 41: pp. 219-224
- 150. Markowitz S.D., et al: Growth stimulation by coexpression of transforming growth factor-alpha and epidermal growth factor-receptor in normal and adenomatous human colon epithelium. J Clin Invest 1990; 86: pp. 356-362
- 151. Liu C., Woo A., and Tsao M.S.: Expression of transforming growth factor-alpha in primary human colon and lung carcinomas. Br J Cancer 1990; 62: pp. 425-429
- 152. Coffey R.J., Shipley G.D., and Moses H.L.: Production of transforming growth factors by human colon cancer lines. Cancer Res 1986; 46: pp. 1164-1169
- 153. Coffey R.J., et al: Transforming growth factor alpha and beta expression in human colon cancer lines: implications for an autocrine model. Cancer Res 1987; 47: pp. 4590-4594
- 154. Moskal T.L., et al: Serum levels of transforming growth factor alpha in gastrointestinal cancer patients. Cancer Epidemiol Biomarkers Prev 1995; 4: pp. 127-131
- 155. Yewale C., et al: Epidermal growth factor receptor targeting in cancer: a review of trends and strategies. Biomaterials 2013; 34: pp. 8690-8707
- 156. Modjtahedi H., and Essapen S.: Epidermal growth factor receptor inhibitors in cancer treatment: advances, challenges and opportunities. Anticancer Drugs 2009; 20: pp. 851-855
- 157. Meyer D., and Birchmeier C.: Multiple essential functions of neuregulin in development. Nature 1995; 378: pp. 386-390
- 158. Crone S.A., and Lee K.F.: Gene targeting reveals multiple essential functions of the neuregulin signaling system during development of the neuroendocrine and nervous systems. Ann N Y Acad Sci 2002; 971: pp. 547-553
- 159. Zhao W.J.: The expression and localization of neuregulin-1 (Nrg1) in the gastrointestinal system of the rhesus monkey. Folia Histochem Cytobiol 2013; 51: pp. 38-44
- 160. Wang G.X., et al: The brown fat-enriched secreted factor Nrg4 preserves metabolic homeostasis through attenuation of hepatic lipogenesis. Nat Med 2014; 20: pp. 1436-1443
- 161. Meyer D., and Birchmeier C.: Distinct isoforms of neuregulin are expressed in mesenchymal and neuronal cells during mouse development. Proc Natl Acad Sci U S A 1994; 91: pp. 1064-1068
- 162. Falls D.L.: Neuregulins: functions, forms, and signaling strategies. Exp Cell Res 2003; 284: pp. 14-30
- 163. Bernard J.K., et al: Neuregulin-4 is a survival factor for colon epithelial cells both in culture and in vivo. J Biol Chem 2012; 287: pp. 39850-39858
- 164. Hayes N.V., et al: Characterization of the cell membrane-associated products of the Neuregulin 4 gene. Oncogene 2008; 27: pp. 715-720
- 165. Hayes N.V., et al: Expression of neuregulin 4 splice variants in normal human tissues and prostate cancer and their effects on cell motility. Endocr Relat Cancer 2011; 18: pp. 39-49
- 166. Harari D., et al: Neuregulin-4: a novel growth factor that acts through the ErbB-4 receptor tyrosine kinase. Oncogene 1999; 18: pp. 2681-2689
- 167. Frey M.R., et al: ErbB4 promotes cyclooxygenase-2 expression and cell survival in colon epithelial cells. Lab Invest 2010; 90: pp. 1415-1424
- 168. Keely S.J., and Barrett K.E.: ErbB2 and ErbB3 receptors mediate inhibition of calcium-dependent chloride secretion in colonic epithelial cells. J Biol Chem 1999; 274: pp. 33449-33454
- 169. Pu J., et al: Neuregulin 1 is involved in enteric nervous system development in zebrafish. J Pediatr Surg 2017; 52: pp. 1182-1187
- 170. Almohazey D., et al: The ErbB3 receptor tyrosine kinase negatively regulates Paneth cells by PI3K-dependent suppression of Atoh1. Cell Death Differ 2017; 24: pp. 855-865
- 171. Paatero I., et al: Interaction with ErbB4 promotes hypoxia-inducible factor-1alpha signaling. J Biol Chem 2012; 287: pp. 9659-9671
- 172. Robinson A., et al: Mucosal protection by hypoxia-inducible factor prolyl hydroxylase inhibition. Gastroenterology 2008; 134: pp. 145-155
- 173. Cummins E.P., et al: The hydroxylase inhibitor dimethyloxalylglycine is protective in a murine model of colitis. Gastroenterology 2008; 134: pp. 156-165
- 174. Pfeifer A.: NRG4: an endocrine link between brown adipose tissue and liver. Cell Metab 2015; 21: pp. 13-14
- 175. Robles A.I., et al: Whole-exome sequencing analyses of inflammatory bowel disease-associated colorectal cancers. Gastroenterology 2016; 150: pp. 931-943
- 176. Tang C.S., et al: Mutations in the NRG1 gene are associated with Hirschsprung disease. Hum Genet 2012; 131: pp. 67-76
- 177. Li Q., et al: Cumulative risk impact of RET, SEMA3, and NRG1 polymorphisms associated with hirschsprung disease in Han Chinese. J Pediatr Gastroenterol Nutr 2017; 64: pp. 385-390
- 178. Yang J., et al: Exome sequencing identified NRG3 as a novel susceptible gene of Hirschsprung’s disease in a Chinese population. Mol Neurobiol 2013; 47: pp. 957-966
- 179. Tang C.S., et al: Genome-wide copy number analysis uncovers a new HSCR gene: NRG3. PLoS Genet 2012; 8: pp. e1002687
- 180. Nagaoka T., et al: Downregulation of epidermal growth factor receptor family receptors and ligands in a mutant K-ras group of patients with colorectal cancer. Mol Med Rep 2016; 13: pp. 3514-3520
- 181. Higashiyama S., et al: A heparin-binding growth factor secreted by macrophage-like cells that is related to EGF. Science 1991; 251: pp. 936-939
- 182. Yoshizumi M., et al: Tumor necrosis factor increases transcription of the heparin-binding epidermal growth factor-like growth factor gene in vascular endothelial cells. J Biol Chem 1992; 267: pp. 9467-9469
- 183. Temizer D.H., et al: Induction of heparin-binding epidermal growth factor-like growth factor mRNA by phorbol ester and angiotensin II in rat aortic smooth muscle cells. J Biol Chem 1992; 267: pp. 24892-24896
- 184. Mulligan C., et al: Microarray analysis of insulin and insulin-like growth factor-1 (IGF-1) receptor signaling reveals the selective up-regulation of the mitogen heparin-binding EGF-like growth factor by IGF-1. J Biol Chem 2002; 277: pp. 42480-42487
- 185. Miyazaki Y., et al: Gastrin induces heparin-binding epidermal growth factor-like growth factor in rat gastric epithelial cells transfected with gastrin receptor. Gastroenterology 1999; 116: pp. 78-89
- 186. Miyazaki Y., et al: Epidermal growth factor receptor mediates stress-induced expression of its ligands in rat gastric epithelial cells. Gastroenterology 2001; 120: pp. 108-116
- 187. McCarthy S.A., et al: Rapid induction of heparin-binding epidermal growth factor/diphtheria toxin receptor expression by Raf and Ras oncogenes. Genes Dev 1995; 9: pp. 1953-1964
- 188. Dluz S.M., et al: Heparin-binding epidermal growth factor-like growth factor expression in cultured fetal human vascular smooth muscle cells. Induction of mRNA levels and secretion of active mitogen. J Biol Chem 1993; 268: pp. 18330-18334
- 189. Bulus N., and Barnard J.A.: Heparin binding epidermal growth factor-like growth factor is a transforming growth factor beta-regulated gene in intestinal epithelial cells. Biochem Biophys Res Commun 1999; 264: pp. 808-812
- 190. Izumi Y., et al: A metalloprotease-disintegrin, MDC9/meltrin-gamma/ ADAM9 and PKCdelta are involved in TPA-induced ectodomain shedding of membrane-anchored heparin-binding EGF-like growth factor. EMBO J 1998; 17: pp. 7260-7272
- 191. Raab G., and Klagsbrun M.: Heparin-binding EGF-like growth factor. Biochim Biophys Acta 1997; 1333: pp. F179-F199
- 192. Elenius K., et al: Activation of HER4 by heparin-binding EGF-like growth factor stimulates chemotaxis but not proliferation. EMBO J 1997; 16: pp. 1268-1278
- 193. Edwards J.P., Zhang X., and Mosser D.M.: The expression of heparin-binding epidermal growth factor-like growth factor by regulatory macrophages. J Immunol 2009; 182: pp. 1929-1939
- 194. Rigo A., et al: Macrophages may promote cancer growth via a GM-CSF/HB-EGF paracrine loop that is enhanced by CXCL12. Mol Cancer 2010; 9: pp. 273
- 195. Nishi E., and Klagsbrun M.: Heparin-binding epidermal growth factor-like growth factor (HB-EGF) is a mediator of multiple physiological and pathological pathways. Growth Factors 2004; 22: pp. 253-260
- 196. Pillai S.B., Turman M.A., and Besner G.E.: Heparin-binding EGF-like growth factor is cytoprotective for intestinal epithelial cells exposed to hypoxia. J Pediatr Surg 1998; 33: pp. 973-978
- 197. Pillai S.B., et al: Heparin-binding epidermal growth factor-like growth factor protects rat intestine from ischemia/reperfusion injury. J Surg Res 1999; 87: pp. 225-231
- 198. Mehta V.B., and Besner G.E.: Inhibition of NF-kappa B activation and its target genes by heparin-binding epidermal growth factor-like growth factor. J Immunol 2003; 171: pp. 6014-6022
- 199. Takemura T., et al: The membrane-bound form of heparin-binding epidermal growth factor-like growth factor promotes survival of cultured renal epithelial cells. J Biol Chem 1997; 272: pp. 31036-31042
- 200. Miyoshi E., et al: Membrane-anchored heparin-binding epidermal growth factor-like growth factor acts as a tumor survival factor in a hepatoma cell line. J Biol Chem 1997; 272: pp. 14349-14355
- 201. Michalsky M.P., et al: Heparin-binding EGF-like growth factor decreases apoptosis in intestinal epithelial cells in vitro. J Pediatr Surg 2001; 36: pp. 1130-1135
- 202. Feng J., El-Assal O.N., and Besner G.E.: Heparin-binding epidermal growth factor-like growth factor reduces intestinal apoptosis in neonatal rats with necrotizing enterocolitis. J Pediatr Surg 2006; 41: pp. 742-747
- 203. Radulescu A., et al: Heparin-binding epidermal growth factor-like growth factor overexpression in transgenic mice increases resistance to necrotizing enterocolitis. J Pediatr Surg 2010; 45: pp. 1933-1939
- 204. Radulescu A., et al: Deletion of the heparin-binding epidermal growth factor-like growth factor gene increases susceptibility to necrotizing enterocolitis. J Pediatr Surg 2010; 45: pp. 729-734
- 205. Yu X., et al: Heparin-binding EGF-like growth factor increases intestinal microvascular blood flow in necrotizing enterocolitis. Gastroenterology 2009; 137: pp. 221-230
- 206. Wei J., and Besner G.E.: M1 to M2 macrophage polarization in heparin-binding epidermal growth factor-like growth factor therapy for necrotizing enterocolitis. J Surg Res 2015; 197: pp. 126-138
- 207. Shoyab M., et al: Amphiregulin: a bifunctional growth-modulating glycoprotein produced by the phorbol 12-myristate 13-acetate-treated human breast adenocarcinoma cell line MCF-7. Proc Natl Acad Sci U S A 1988; 85: pp. 6528-6532
- 208. Nishimura T., et al: Amphiregulin and epiregulin expression in neoplastic and inflammatory lesions in the colon. Oncol Rep 2008; 19: pp. 105-110
- 209. Saeki T., et al: Differential immunohistochemical detection of amphiregulin and cripto in human normal colon and colorectal tumors. Cancer Res 1992; 52: pp. 3467-3473
- 210. Plowman G.D., et al: The amphiregulin gene encodes a novel epidermal growth factor-related protein with tumor-inhibitory activity. Mol Cell Biol 1990; 10: pp. 1969-1981
- 211. Johnson G.R., et al: Autocrine action of amphiregulin in a colon carcinoma cell line and immunocytochemical localization of amphiregulin in human colon. J Cell Biol 1992; 118: pp. 741-751
- 212. De Angelis E., et al: Expression of cripto and amphiregulin in colon mucosa from high risk colon cancer families. Int J Oncol 1999; 14: pp. 437-440
- 213. Ciardiello F., et al: Differential expression of epidermal growth factor- related proteins in human colorectal tumors. Proc Natl Acad Sci U S A 1991; 88: pp. 7792-7796
- 214. Shoyab M., et al: Structure and function of human amphiregulin: a member of the epidermal growth factor family. Science 1989; 243: pp. 1074-1076
- 215. Normanno N., et al: Amphiregulin anti-sense oligodeoxynucleotides inhibit growth and transformation of a human colon carcinoma cell line. Int J Cancer 1995; 62: pp. 762-766
- 216. Damstrup L., et al: Amphiregulin acts as an autocrine growth factor in two human polarizing colon cancer lines that exhibit domain selective EGF receptor mitogenesis. Br J Cancer 1999; 80: pp. 1012-1019
- 217. Higginbotham J.N., et al: Amphiregulin exosomes increase cancer cell invasion. Curr Biol 2011; 21: pp. 779-786
- 218. Toyoda H., et al: Epiregulin. A novel epidermal growth factor with mitogenic activity for rat primary hepatocytes. J Biol Chem 1995; 270: pp. 7495-7500
- 219. Shirakata Y., et al: Epiregulin, a novel member of the epidermal growth factor family, is an autocrine growth factor in normal human keratinocytes. J Biol Chem 2000; 275: pp. 5748-5753
- 220. Riese D.J., and Cullum R.L.: Epiregulin: roles in normal physiology and cancer. Semin Cell Dev Biol 2014; 28: pp. 49-56
- 221. Toyoda H., et al: Distribution of mRNA for human epiregulin, a differentially expressed member of the epidermal growth factor family. Biochem J 1997; 326: pp. 69-75
- 222. Neufert C., et al: Tumor fibroblast-derived epiregulin promotes growth of colitis-associated neoplasms through ERK. J Clin Invest 2013; 123: pp. 1428-1443
- 223. Gregorieff A., et al: Yap-dependent reprogramming of Lgr5(+) stem cells drives intestinal regeneration and cancer. Nature 2015; 526: pp. 715-718
- 224. Lee D., et al: Epiregulin is not essential for development of intestinal tumors but is required for protection from intestinal damage. Mol Cell Biol 2004; 24: pp. 8907-8916
- 225. Shing Y., et al: Betacellulin: a mitogen from pancreatic beta cell tumors. Science 1993; 259: pp. 1604-1607
- 226. Kallincos N.C., et al: Cloning of rat betacellulin and characterization of its expression in the gastrointestinal tract. Growth Factors 2000; 18: pp. 203-213
- 227. Dunbar A.J., et al: Identification of betacellulin as a major peptide growth factor in milk: purification, characterization and molecular cloning of bovine betacellulin. Biochem J 1999; 344: pp. 713-721
- 228. Bastian S.E., et al: Measurement of betacellulin levels in bovine serum, colostrum and milk. J Endocrinol 2001; 168: pp. 203-212
- 229. Saito T., et al: Differential activation of epidermal growth factor (EGF) receptor downstream signaling pathways by betacellulin and EGF. Endocrinology 2004; 145: pp. 4232-4243
- 230. Dahlhoff M., et al: Betacellulin stimulates growth of the mouse intestinal epithelium and increases adenoma multiplicity in Apc . FEBS Lett 2008; 582: pp. 2911-2915
- 231. Howarth G.S., et al: Betacellulin promotes growth of the gastrointestinal organs and effects a diuresis in normal rats. Growth Factors 2003; 21: pp. 79-86
- 232. Strachan L., et al: Cloning and biological activity of epigen, a novel member of the epidermal growth factor superfamily. J Biol Chem 2001; 276: pp. 18265-18271
- 233. de Larco J.E., and Todaro G.J.: Growth factors from murine sarcoma virus-transformed cells. Proc Natl Acad Sci U S A 1978; 75: pp. 4001-4005
- 234. Kawabata M., Imamura T., and Miyazono K.: Signal transduction by bone morphogenetic proteins. Cytokine Growth Factor Rev 1998; 9: pp. 49-61
- 235. Zhao G.Q.: Consequences of knocking out BMP signaling in the mouse. Genesis 2003; 35: pp. 43-56
- 236. Aleman-Muench G.R., and Soldevila G.: When versatility matters: activins/inhibins as key regulators of immunity. Immunol Cell Biol 2012; 90: pp. 137-148
- 237. Mather J.P., Moore A., and Li R.H.: Activins, inhibins, and follistatins: further thoughts on a growing family of regulators. Proc Soc Exp Biol Med 1997; 215: pp. 209-222
- 238. Schier A.F.: Nodal signaling in vertebrate development. Annu Rev Cell Dev Biol 2003; 19: pp. 589-621
- 239. Hoffmann F.M.: TGF-beta family factors in Drosophila morphogenesis. Mol Reprod Dev 1992; 32: pp. 173-178
- 240. Lane A.H., and Donahoe P.K.: New insights into mullerian inhibiting substance and its mechanism of action. J Endocrinol 1998; 158: pp. 1-6
- 241. Heine U.I., et al: Localization of transforming growth factor-beta 1 in mitochondria of murine heart and liver. Cell Regul 1991; 2: pp. 467-477
- 242. Shi Y., and Massague J.: Mechanisms of TGF-beta signaling from cell membrane to the nucleus. Cell 2003; 113: pp. 685-700
- 243. Barnard J.A., et al: Regulation of intestinal epithelial cell growth by transforming growth factor type beta. Proc Natl Acad Sci U S A 1989; 86: pp. 1578-1582
- 244. Glick A.B., et al: Complex regulation of TGF beta expression by retinoic acid in the vitamin A-deficient rat. Development 1991; 111: pp. 1081-1086
- 245. Avery A., et al: TGF-beta expression in the human colon: differential immunostaining along crypt epithelium. Br J Cancer 1993; 68: pp. 137-139
- 246. Barnard J.A., Warwick G.J., and Gold L.I.: Localization of transforming growth factor beta isoforms in the normal murine small intestine and colon. Gastroenterology 1993; 105: pp. 67-73
- 247. Babyatsky M.W., Rossiter G., and Podolsky D.K.: Expression of transforming growth factors alpha and beta in colonic mucosa in inflammatory bowel disease. Gastroenterology 1996; 110: pp. 975-984
- 248. Dignass A.U., Stow J.L., and Babyatsky M.W.: Acute epithelial injury in the rat small intestine in vivo is associated with expanded expression of transforming growth factor alpha and beta. Gut 1996; 38: pp. 687-693
- 249. Van Laethem J.L., et al: Localization of transforming growth factor-beta 1 precursor and latent TGF-beta 1 binding protein in colorectal adenomas. Dig Dis Sci 1996; 41: pp. 1741-1748
- 250. Ruifrok A.C., et al: Spatial and temporal patterns of expression of epidermal growth factor, transforming growth factor alpha and transforming growth factor beta 1-3 and their receptors in mouse jejunum after radiation treatment. Radiat Res 1997; 147: pp. 1-12
- 251. Shao J., et al: Coordinate regulation of cyclooxygenase-2 and TGF-beta1 in replication error-positive colon cancer and azoxymethane-induced rat colonic tumors. Carcinogenesis 1999; 20: pp. 185-191
- 252. Dunker N., et al: The role of transforming growth factor beta-2, beta-3 in mediating apoptosis in the murine intestinal mucosa. Gastroenterology 2002; 122: pp. 1364-1375
- 253. Qian S.W., et al: Binding affinity of transforming growth factor-beta for its type II receptor is determined by the C-terminal region of the molecule. J Biol Chem 1996; 271: pp. 30656-30662
- 254. Gorvy D.A., et al: Experimental manipulation of transforming growth factor-beta isoforms significantly affects adhesion formation in a murine surgical model. Am J Pathol 2005; 167: pp. 1005-1019
- 255. Yue J., and Mulder K.M.: Transforming growth factor-beta signal transduction in epithelial cells. Pharmacol Ther 2001; 91: pp. 1-34
- 256. Yu L., Hebert M.C., and Zhang Y.E.: TGF-beta receptor-activated p38 MAP kinase mediates Smad-independent TGF-beta responses. EMBO J 2002; 21: pp. 3749-3759
- 257. Pertovaara L., et al: Enhanced jun gene expression is an early genomic response to transforming growth factor beta stimulation. Mol Cell Biol 1989; 9: pp. 1255-1262
- 258. Pietenpol J.A., et al: TGF-beta 1 inhibition of c-myc transcription and growth in keratinocytes is abrogated by viral transforming proteins with pRB binding domains. Cell 1990; 61: pp. 777-785
- 259. Lee K.Y., and Bae S.C.: TGF-beta-dependent cell growth arrest and apoptosis. J Biochem Mol Biol 2002; 35: pp. 47-53
- 260. Mithani S.K., et al: Smad3 has a critical role in TGF-beta-mediated growth inhibition and apoptosis in colonic epithelial cells. J Surg Res 2004; 117: pp. 296-305
- 261. Wang C.Y., et al: Both transforming growth factor-beta and substrate release are inducers of apoptosis in a human colon adenoma cell line. Cancer Res 1995; 55: pp. 5101-5105
- 262. Derynck R., Akhurst R.J., and Balmain A.: TGF-beta signaling in tumor suppression and cancer progression. Nat Genet 2001; 29: pp. 117-129
- 263. Tsushima H., et al: Circulating transforming growth factor beta 1 as a predictor of liver metastasis after resection in colorectal cancer. Clin Cancer Res 2001; 7: pp. 1258-1262
- 264. Picon A., et al: A subset of metastatic human colon cancers expresses elevated levels of transforming growth factor beta1. Cancer Epidemiol Biomarkers Prev 1998; 7: pp. 497-504
- 265. Oft M., Heider K.H., and Beug H.: TGFbeta signaling is necessary for carcinoma cell invasiveness and metastasis. Curr Biol 1998; 8: pp. 1243-1252
- 266. Larsson J., et al: Abnormal angiogenesis but intact hematopoietic potential in TGF-beta type I receptor-deficient mice. EMBO J 2001; 20: pp. 1663-1673
- 267. Daniels C.E., et al: Imatinib mesylate inhibits the profibrogenic activity of TGF-beta and prevents bleomycin-mediated lung fibrosis. J Clin Invest 2004; 114: pp. 1308-1316
- 268. Friedman E., et al: High levels of transforming growth factor beta 1 correlate with disease progression in human colon cancer. Cancer Epidemiol Biomarkers Prev 1995; 4: pp. 549-554
- 269. Huang A., et al: Increased serum transforming growth factor-beta1 in human colorectal cancer correlates with reduced circulating dendritic cells and increased colonic Langerhans cell infiltration. Clin Exp Immunol 2003; 134: pp. 270-278
- 270. Hsu S., et al: Colon carcinoma cells switch their response to transforming growth factor beta 1 with tumor progression. Cell Growth Differ 1994; 5: pp. 267-275
- 271. Deane N.G., et al: Enhanced tumor formation in cyclin D1 x transforming growth factor beta1 double transgenic mice with characterization by magnetic resonance imaging. Cancer Res 2004; 64: pp. 1315-1322
- 272. Geiser A.G., et al: Characterization of the mouse transforming growth factor-beta 1 promoter and activation by the Ha-ras oncogene. Mol Cell Biol 1991; 11: pp. 84-92
- 273. Mishra L., et al: The role of TGF-beta and Wnt signaling in gastrointestinal stem cells and cancer. Oncogene 2005; 24: pp. 5775-5789
- 274. Hauck A.L., et al: Twists and turns in the development and maintenance of the mammalian small intestine epithelium. Birth Defects Res C Embryo Today 2005; 75: pp. 58-71
- 275. Monteleone G., et al: Blocking Smad7 restores TGF-beta1 signaling in chronic inflammatory bowel disease. J Clin Invest 2001; 108: pp. 601-609
- 276. Beck P.L., et al: Transforming growth factor-beta mediates intestinal healing and susceptibility to injury in vitro and in vivo through epithelial cells. Am J Pathol 2003; 162: pp. 597-608
- 277. Dickson M.C., et al: Defective haematopoiesis and vasculogenesis in transforming growth factor-beta 1 knock out mice. Development 1995; 121: pp. 1845-1854
- 278. Shull M.M., et al: Targeted disruption of the mouse transforming growth factor-beta 1 gene results in multifocal inflammatory disease. Nature 1992; 359: pp. 693-699
- 279. Kulkarni A.B., et al: Transforming growth factor beta 1 null mutation in mice causes excessive inflammatory response and early death. Proc Natl Acad Sci U S A 1993; 90: pp. 770-774
- 280. Boivin G.P., et al: Germ-free and barrier-raised TGF beta 1-deficient mice have similar inflammatory lesions. Transgenic Res 1997; 6: pp. 197-202
- 281. Proetzel G., et al: Transforming growth factor-beta 3 is required for secondary palate fusion. Nat Genet 1995; 11: pp. 409-414
- 282. Sanford L.P., et al: TGFbeta2 knockout mice have multiple developmental defects that are non-overlapping with other TGFbeta knockout phenotypes. Development 1997; 124: pp. 2659-2670
- 283. Letterio J.J., and Roberts A.B.: TGF-beta: a critical modulator of immune cell function. Clin Immunol Immunopathol 1997; 84: pp. 244-250
- 284. McGeachy M.J., et al: TGF-beta and IL-6 drive the production of IL-17 and IL-10 by T cells and restrain T(H)-17 cell-mediated pathology. Nat Immunol 2007; 8: pp. 1390-1397
- 285. Hannigan M., et al: The role of p38 MAP kinase in TGF-beta1-induced signal transduction in human neutrophils. Biochem Biophys Res Commun 1998; 246: pp. 55-58
- 286. Cazac B.B., and Roes J.: TGF-beta receptor controls B cell responsiveness and induction of IgA in vivo. Immunity 2000; 13: pp. 443-451
- 287. Gebhardt T., et al: Growth, phenotype, and function of human intestinal mast cells are tightly regulated by transforming growth factor beta1. Gut 2005; 54: pp. 928-934
- 288. Urist M.R.: Bone morphogenetic protein: the molecularization of skeletal system development. J Bone Miner Res 1997; 12: pp. 343-346
- 289. De Santa Barbara P., et al: Bone morphogenetic protein signaling pathway plays multiple roles during gastrointestinal tract development. Dev Dyn 2005; 234: pp. 312-322
- 290. Batts L.E., et al: Bmp signaling is required for intestinal growth and morphogenesis. Dev Dyn 2006; 235: pp. 1563-1570
- 291. McCracken K.W., et al: Modelling human development and disease in pluripotent stem-cell-derived gastric organoids. Nature 2014; 516: pp. 400-404
- 292. Wang R.N., et al: Bone morphogenetic protein (BMP) signaling in development and human diseases. Genes Dis 2014; 1: pp. 87-105
- 293. He X.C., et al: BMP signaling inhibits intestinal stem cell self-renewal through suppression of Wnt-beta-catenin signaling. Nat Genet 2004; 36: pp. 1117-1121
- 294. Sato T., et al: Single Lgr5 stem cells build crypt-villus structures in vitro without a mesenchymal niche. Nature 2009; 459: pp. 262-265
- 295. Maric I., et al: Bone morphogenetic protein-7 reduces the severity of colon tissue damage and accelerates the healing of inflammatory bowel disease in rats. J Cell Physiol 2003; 196: pp. 258-264
- 296. Wang L., et al: The bone morphogenetic protein-hepcidin axis as a therapeutic target in inflammatory bowel disease. Inflamm Bowel Dis 2012; 18: pp. 112-119
- 297. Milano F., et al: Bone morphogenetic protein 4 expressed in esophagitis induces a columnar phenotype in esophageal squamous cells. Gastroenterology 2007; 132: pp. 2412-2421
- 298. Jiang M., et al: BMP-driven NRF2 activation in esophageal basal cell differentiation and eosinophilic esophagitis. J Clin Invest 2015; 125: pp. 1557-1568
- 299. Castillo D., et al: Activation of the BMP4 pathway and early expression of CDX2 characterize non-specialized columnar metaplasia in a human model of Barretťs esophagus. J Gastrointest Surg 2012; 16: pp. 227-237
- 300. Zhang Y., et al: Bone morphogenetic protein 2 inhibits the proliferation and growth of human colorectal cancer cells. Oncol Rep 2014; 32: pp. 1013-1020
- 301. Yuvaraj S., et al: [object Object]. Gastroenterol Res Pract 2012; 2012: pp. 895462
- 302. Beck S.E., et al: Bone morphogenetic protein signaling and growth suppression in colon cancer. Am J Physiol Gastrointest Liver Physiol 2006; 291: pp. G135-G145
- 303. Kodach L.L., et al: The bone morphogenetic protein pathway is active in human colon adenomas and inactivated in colorectal cancer. Cancer 2008; 112: pp. 300-306
- 304. Lorente-Trigos A., et al: BMP signaling promotes the growth of primary human colon carcinomas in vivo. J Mol Cell Biol 2010; 2: pp. 318-332
- 305. Wildi S., et al: Overexpression of activin A in stage IV colorectal cancer. Gut 2001; 49: pp. 409-417
- 306. Dignass A.U., et al: Functional relevance of activin A in the intestinal epithelium. Scand J Gastroenterol 2002; 37: pp. 936-943
- 307. Fukamachi H., et al: Activin A regulates growth of gastro-intestinal epithelial cells by mediating epithelial-mesenchymal interaction. Dev Growth Differ 2013; 55: pp. 786-791
- 308. Hubner G., et al: Activin A: a novel player and inflammatory marker in inflammatory bowel disease? Lab Invest 1997; 77: pp. 311-318
- 309. Zhang Y.Q., et al: Upregulation of activin signaling in experimental colitis. Am J Physiol Gastrointest Liver Physiol 2009; 297: pp. G768-G780
- 310. Refaat B., et al: Activins and their related proteins in colon carcinogenesis: insights from early and advanced azoxymethane rat models of colon cancer. BMC Cancer 2016; 16: pp. 879
- 311. Donovan S.M., Hintz R.L., and Rosenfeld R.G.: Insulin-like growth factors I and II and their binding proteins in human milk: effect of heat treatment on IGF and IGF binding protein stability. J Pediatr Gastroenterol Nutr 1991; 13: pp. 242-253
- 312. Eriksson U., et al: Insulin-like growth factors (IGF) I and II and IGF binding proteins (IGFBPs) in human colostrum/transitory milk during the first week postpartum: comparison with neonatal and maternal serum. Biochem Biophys Res Commun 1993; 196: pp. 267-273
- 313. Chaurasia O.P., Marcuard S.P., and Seidel E.R.: Insulin-like growth factor I in human gastrointestinal exocrine secretions. Regul Pept 1994; 50: pp. 113-119
- 314. Cui H., et al: Loss of IGF2 imprinting: a potential marker of colorectal cancer risk. Science 2003; 299: pp. 1753-1755
- 315. Cheng Y.W., et al: Loss of imprinting and marked gene elevation are 2 forms of aberrant IGF2 expression in colorectal cancer. Int J Cancer 2010; 127: pp. 568-577
- 316. Rechler M.M., and Nissley S.P.: Insulin-like growth factor (IGF)/somatomedin receptor subtypes: structure, function, and relationships to insulin receptors and IGF carrier proteins. Horm Res 1986; 24: pp. 152-159
- 317. Izumi T., et al: Insulin-like growth factor I rapidly stimulates tyrosine phosphorylation of a Mr 185,000 protein in intact cells. J Biol Chem 1987; 262: pp. 1282-1287
- 318. Kuemmerle J.F., and Murthy K.S.: Coupling of the insulin-like growth factor-I receptor tyrosine kinase to Gi2 in human intestinal smooth muscle: Gbetagamma-dependent mitogen-activated protein kinase activation and growth. J Biol Chem 2001; 276: pp. 7187-7194
- 319. Firth S.M., and Baxter R.C.: Cellular actions of the insulin-like growth factor binding proteins. Endocr Rev 2002; 23: pp. 824-854
- 320. Shoubridge C.A., Steeb C.B., and Read L.C.: IGFBP mRNA expression in small intestine of rat during postnatal development. Am J Physiol Gastrointest Liver Physiol 2001; 281: pp. G1378-G1384
- 321. Winesett D.E., et al: Regulation and localization of the insulin-like growth factor system in small bowel during altered nutrient status. Am J Physiol 1995; 268: pp. G631-G640
- 322. Wick D.A., Seetharam B., and Dahms N.M.: Basolateral sorting signal of the 300-kDa mannose 6-phosphate receptor. Am J Physiol Gastrointest Liver Physiol 2002; 282: pp. G51-G60
- 323. Dahms N.M., Seetharam B., and Wick D.A.: Expression of insulin-like growth factor (IGF)-I receptors, IGF-II/cation-independent mannose 6-phosphate receptors (CI-MPRs), and cation-dependent MPRs in polarized human intestinal Caco-2 cells. Biochim Biophys Acta 1996; 1279: pp. 84-92
- 324. Liu J.P., et al: Mice carrying null mutations of the genes encoding insulin-like growth factor I (Igf-1) and type 1 IGF receptor (Igf1r). Cell 1993; 75: pp. 59-72
- 325. Butler A.A., and LeRoith D.: Minireview: tissue-specific versus generalized gene targeting of the igf1 and igf1r genes and their roles in insulin-like growth factor physiology. Endocrinology 2001; 142: pp. 1685-1688
- 326. Wang J., et al: Targeted overexpression of IGF-I evokes distinct patterns of organ remodeling in smooth muscle cell tissue beds of transgenic mice. J Clin Invest 1997; 100: pp. 1425-1439
- 327. Ohneda K., et al: Enhanced growth of small bowel in transgenic mice expressing human insulin-like growth factor I. Gastroenterology 1997; 112: pp. 444-454
- 328. Steeb C.B., Trahair J.F., and Read L.C.: Administration of insulin-like growth factor-I (IGF-I) peptides for three days stimulates proliferation of the small intestinal epithelium in rats. Gut 1995; 37: pp. 630-638
- 329. Steeb C.B., et al: Prolonged administration of IGF peptides enhances growth of gastrointestinal tissues in normal rats. Am J Physiol 1994; 266: pp. G1090-G1098
- 330. Mahavadi S., et al: Amelioration of excess collagen IalphaI, fibrosis, and smooth muscle growth in TNBS-induced colitis in IGF-I(+/-) mice. Inflamm Bowel Dis 2011; 17: pp. 711-719
- 331. Liu S., et al: Recombinant . BMC Biotechnol 2016; 16: pp. 25
- 332. Burrin D.G., et al: Orally administered IGF-I increases intestinal mucosal growth in formula-fed neonatal pigs. Am J Physiol 1996; 270: pp. R1085-R1091
- 333. Wilkins H.R., et al: Reduction of spontaneous and irradiation-induced apoptosis in small intestine of IGF-I transgenic mice. Am J Physiol Gastrointest Liver Physiol 2002; 283: pp. G457-G464
- 334. Mathews L.S., et al: Growth enhancement of transgenic mice expressing human insulin-like growth factor I. Endocrinology 1988; 123: pp. 2827-2833
- 335. Potten C.S., et al: Stimulation and inhibition of proliferation in the small intestinal crypts of the mouse after in vivo administration of growth factors. Gut 1995; 36: pp. 864-873
- 336. Trahair J.F., et al: Regulation of gastrointestinal growth in fetal sheep by luminally administered insulin-like growth factor-I. J Endocrinol 1997; 152: pp. 29-38
- 337. Vanderhoof J.A., et al: Truncated and native insulinlike growth factor I enhance mucosal adaptation after jejunoileal resection. Gastroenterology 1992; 102: pp. 1949-1956
- 338. Mantell M.P., et al: Resection-induced colonic adaptation is augmented by IGF-I and associated with upregulation of colonic IGF-I mRNA. Am J Physiol 1995; 269: pp. G974-G980
- 339. Dahly E.M., Guo Z., and Ney D.M.: IGF-I augments resection-induced mucosal hyperplasia by altering enterocyte kinetics. Am J Physiol Regul Integr Comp Physiol 2003; 285: pp. R800-R808
- 340. Zhang W., et al: Insulin-like growth factor-I improves mucosal structure and function in transplanted rat small intestine. Transplantation 1995; 59: pp. 755-761
- 341. Peterson C.A., et al: Beneficial effects of insulin-like growth factor I on epithelial structure and function in parenterally fed rat jejunum. Gastroenterology 1996; 111: pp. 1501-1508
- 342. Schall K.A., et al: Adult zebrafish intestine resection: a novel model of short bowel syndrome, adaptation, and intestinal stem cell regeneration. Am J Physiol Gastrointest Liver Physiol 2015; 309: pp. G135-G145
- 343. Inaba T., et al: Insulin-like growth factor 1 has beneficial effects, whereas growth hormone has limited effects on postoperative protein metabolism, gut integrity, and splenic weight in rats with chronic mild liver injury. JPEN J Parenter Enteral Nutr 1997; 21: pp. 55-62
- 344. Chen K., et al: Insulin-like growth factor-I prevents gut atrophy and maintains intestinal integrity in septic rats. JPEN J Parenter Enteral Nutr 1995; 19: pp. 119-124
- 345. Wolpin B.M., et al: Insulin, the insulin-like growth factor axis, and mortality in patients with nonmetastatic colorectal cancer. J Clin Oncol 2009; 27: pp. 176-185
- 346. Sridhar S.S., and Goodwin P.J.: Insulin-insulin-like growth factor axis and colon cancer. J Clin Oncol 2009; 27: pp. 165-167
- 347. Nosho K., et al: Interplay of insulin-like growth factor-II, insulin- like growth factor-I, insulin-like growth factor-I receptor, COX-2, and matrix metalloproteinase-7, play key roles in the early stage of colorectal carcinogenesis. Clin Cancer Res 2004; 10: pp. 7950-7957
- 348. Vigneri P.G., et al: The insulin/IGF system in colorectal cancer development and resistance to therapy. Front Oncol 2015; 5: pp. 230
- 349. D’Addio F., et al: Circulating IGF-I and IGFBP3 levels control human colonic stem cell function and are disrupted in diabetic enteropathy. Cell Stem Cell 2015; 17: pp. 486-498
- 350. Flynn R.S., et al: Endogenous IGF-I and alphaVbeta3 integrin ligands regulate increased smooth muscle hyperplasia in stricturing Crohn’s disease. Gastroenterology 2010; 138: pp. 285-293
- 351. Gupta N., et al: Sex differences in statural growth impairment in Crohn’s disease: role of IGF-1. Inflamm Bowel Dis 2011; 17: pp. 2318-2325
- 352. Katsanos K.H., et al: Reduced serum insulin-like growth factor-1 (IGF-1) and IGF-binding protein-3 levels in adults with inflammatory bowel disease. Growth Horm IGF Res 2001; 11: pp. 364-367
- 353. Russell W.E., McGowan J.A., and Bucher N.L.: Partial characterization of a hepatocyte growth factor from rat platelets. J Cell Physiol 1984; 119: pp. 183-192
- 354. Nakamura T., Nawa K., and Ichihara A.: Partial purification and characterization of hepatocyte growth factor from serum of hepatectomized rats. Biochem Biophys Res Commun 1984; 122: pp. 1450-1459
- 355. Michalopoulos G., et al: Control of hepatocyte replication by two serum factors. Cancer Res 1984; 44: pp. 4414-4419
- 356. Tamura M., et al: Enhancement of human hepatocyte growth factor production by interleukin-1 alpha and -1 beta and tumor necrosis factor-alpha by fibroblasts in culture. J Biol Chem 1993; 268: pp. 8140-8145
- 357. Matsumoto K., Okazaki H., and Nakamura T.: Up-regulation of hepatocyte growth factor gene expression by interleukin-1 in human skin fibroblasts. Biochem Biophys Res Commun 1992; 188: pp. 235-243
- 358. Gohda E., et al: TGF-beta is a potent inhibitor of hepatocyte growth factor secretion by human fibroblasts. Cell Biol Int Rep 1992; 16: pp. 917-926
- 359. Gohda E., et al: Induction of hepatocyte growth factor in human skin fibroblasts by epidermal growth factor, platelet-derived growth factor and fibroblast growth factor. Cytokine 1994; 6: pp. 633-640
- 360. Takayama H., et al: Ulcerative proctitis, rectal prolapse, and intestinal pseudo-obstruction in transgenic mice overexpressing hepatocyte growth factor/scatter factor. Lab Invest 2001; 81: pp. 297-305
- 361. Stoker M., and Gherardi E.: Regulation of cell movement: the motogenic cytokines. Biochim Biophys Acta 1991; 1072: pp. 81-102
- 362. Rubin J.S., et al: A broad-spectrum human lung fibroblast-derived mitogen is a variant of hepatocyte growth factor. Proc Natl Acad Sci U S A 1991; 88: pp. 415-419
- 363. Rosen E.M., et al: Regulation of scatter factor production via a soluble inducing factor. J Cell Biol 1994; 127: pp. 225-234
- 364. Montesano R., et al: Identification of a fibroblast-derived epithelial morphogen as hepatocyte growth factor. Cell 1991; 67: pp. 901-908
- 365. Nusrat A., et al: Hepatocyte growth factor/scatter factor effects on epithelia. Regulation of intercellular junctions in transformed and nontransformed cell lines, basolateral polarization of c-met receptor in transformed and natural intestinal epithelia, and induction of rapid wound repair in a transformed model epithelium. J Clin Invest 1994; 93: pp. 2056-2065
- 366. Fukamachi H., et al: Hepatocyte growth factor region specifically stimulates gastro-intestinal epithelial growth in primary culture. Biochem Biophys Res Commun 1994; 205: pp. 1445-1451
- 367. Yamada Y., Saito S., and Morikawa H.: Hepatocyte growth factor in human breast milk. Am J Reprod Immunol 1998; 40: pp. 112-120
- 368. Kinoshita T., Tashiro K., and Nakamura T.: Marked increase of HGF mRNA in non-parenchymal liver cells of rats treated with hepatotoxins. Biochem Biophys Res Commun 1989; 165: pp. 1229-1234
- 369. Sunitha I., et al: Hepatocyte growth factor stimulates invasion across reconstituted basement membranes by a new human small intestinal cell line. Clin Exp Metastasis 1994; 12: pp. 143-154
- 370. Jiang W., et al: Hepatocyte growth factor/scatter factor, its molecular, cellular and clinical implications in cancer. Crit Rev Oncol Hematol 1999; 29: pp. 209-248
- 371. Miyazawa K., et al: Molecular cloning and sequence analysis of the cDNA for a human serine protease reponsible for activation of hepatocyte growth factor. Structural similarity of the protease precursor to blood coagulation factor XII. J Biol Chem 1993; 268: pp. 10024-10028
- 372. Kinoshita Y., et al: Production and activation of hepatocyte growth factor during the healing of rat gastric ulcers. Digestion 1997; 58: pp. 225-231
- 373. Itoh H., et al: Mouse hepatocyte growth factor activator gene: its expression not only in the liver but also in the gastrointestinal tract. Biochim Biophys Acta 2000; 1491: pp. 295-302
- 374. Orian-Rousseau V., et al: CD44 is required for two consecutive steps in HGF/c-Met signaling. Genes Dev 2002; 16: pp. 3074-3086
- 375. Ponzetto C., et al: A multifunctional docking site mediates signaling and transformation by the hepatocyte growth factor/scatter factor receptor family. Cell 1994; 77: pp. 261-271
- 376. Ponzetto C., et al: A novel recognition motif for phosphatidylinositol 3-kinase binding mediates its association with the hepatocyte growth factor/scatter factor receptor. Mol Cell Biol 1993; 13: pp. 4600-4608
- 377. Pawson T., and Gish G.D.: SH2 and SH3 domains: from structure to function. Cell 1992; 71: pp. 359-362
- 378. Koch C.A., et al: SH2 and SH3 domains: elements that control interactions of cytoplasmic signaling proteins. Science 1991; 252: pp. 668-674
- 379. Kostyuk P.G., Krishtal O.A., and Shakhovalov Y.A.: Separation of sodium and calcium currents in the somatic membrane of mollusc neurones. J Physiol 1977; 270: pp. 545-568
- 380. Boccaccio C., et al: Hepatocyte growth factor (HGF) receptor expression is inducible and is part of the delayed-early response to HGF. J Biol Chem 1994; 269: pp. 12846-12851
- 381. Chen P., et al: Epidermal growth factor receptor-mediated cell motility: phospholipase C activity is required, but mitogen-activated protein kinase activity is not sufficient for induced cell movement. J Cell Biol 1994; 127: pp. 847-857
- 382. Moghul A., et al: Modulation of c-MET proto-oncogene (HGF receptor) mRNA abundance by cytokines and hormones: evidence for rapid decay of the 8 . Oncogene 1994; 9: pp. 2045-2052
- 383. Kato Y., et al: Hepatocyte growth factor enhances intestinal mucosal cell function and mass in vivo. J Pediatr Surg 1997; 32: pp. 991-994
- 384. Kato Y., et al: Influence of luminal hepatocyte growth factor on small intestine mucosa in vivo. J Surg Res 1997; 71: pp. 49-53
- 385. Kermorgant S., et al: Developmental expression and functionality of hepatocyte growth factor and c-Met in human fetal digestive tissues. Gastroenterology 1997; 112: pp. 1635-1647
- 386. Nishimura S., et al: Hepatocyte growth factor accelerates restitution of intestinal epithelial cells. J Gastroenterol 1998; 33: pp. 172-178
- 387. Takahashi M., et al: Hepatocyte growth factor stimulates wound repair of the rabbit esophageal epithelial cells in primary culture. Biochem Biophys Res Commun 1995; 216: pp. 298-305
- 388. Kolatsi-Joannou M., et al: Expression of hepatocyte growth factor/scatter factor and its receptor, MET, suggests roles in human embryonic organogenesis. Pediatr Res 1997; 41: pp. 657-665
- 389. Avetisyan M., et al: Hepatocyte growth factor and MET support mouse enteric nervous system development, the peristaltic response, and intestinal epithelial proliferation in response to injury. J Neurosci 2015; 35: pp. 11543-11558
- 390. Goke M., Kanai M., and Podolsky D.K.: Intestinal fibroblasts regulate intestinal epithelial cell proliferation via hepatocyte growth factor. Am J Physiol 1998; 274: pp. G809-G818
- 391. Khurana S.S., et al: The hyaluronic acid receptor CD44 coordinates normal and metaplastic gastric epithelial progenitor cell proliferation. J Biol Chem 2013; 288: pp. 16085-16097
- 392. Matsubara Y., et al: Hepatocyte growth factor activator: a possible regulator of morphogenesis during fetal development of the rat gastrointestinal tract. Biochem Biophys Res Commun 1998; 253: pp. 477-484
- 393. Schmidt C., et al: Scatter factor/hepatocyte growth factor is essential for liver development. Nature 1995; 373: pp. 699-702
- 394. Uehara Y., et al: Placental defect and embryonic lethality in mice lacking hepatocyte growth factor/scatter factor. Nature 1995; 373: pp. 702-705
- 395. Watanabe S., et al: Hepatocyte growth factor accelerates the wound repair of cultured gastric mucosal cells. Biochem Biophys Res Commun 1994; 199: pp. 1453-1460
- 396. Itoh H., and Kataoka H.: Roles of hepatocyte growth factor activator (HGFA) and its inhibitor HAI-1 in the regeneration of injured gastrointestinal mucosa. J Gastroenterol 2002; 37: pp. 15-21
- 397. Bertaux-Skeirik N., et al: CD44 variant isoform 9 emerges in response to injury and contributes to the regeneration of the gastric epithelium. J Pathol 2017; 242: pp. 463-475
- 398. Ortega-Cava C.F., et al: Hepatocyte growth factor expression in dextran sodium sulfate-induced colitis in rats. Dig Dis Sci 2002; 47: pp. 2275-2285
- 399. Kitamura S., et al: Expression of hepatocyte growth factor and c-met in ulcerative colitis. Inflamm Res 2000; 49: pp. 320-324
- 400. Tahara Y., et al: Hepatocyte growth factor facilitates colonic mucosal repair in experimental ulcerative colitis in rats. J Pharmacol Exp Ther 2003; 307: pp. 146-151
- 401. Oh K., et al: Ameliorating effect of hepatocyte growth factor on inflammatory bowel disease in a murine model. Am J Physiol Gastrointest Liver Physiol 2005; 288: pp. G729-G735
- 402. Dignass A.U., Lynch-Devaney K., and Podolsky D.K.: Hepatocyte growth factor/scatter factor modulates intestinal epithelial cell proliferation and migration. Biochem Biophys Res Commun 1994; 202: pp. 701-709
- 403. Pasdar M., et al: Inhibition of junction assembly in cultured epithelial cells by hepatocyte growth factor/scatter factor is concomitant with increased stability and altered phosphorylation of the soluble junctional molecules. Cell Growth Differ 1997; 8: pp. 451-462
- 404. Tannapfel A., et al: Effect of hepatocyte growth factor on the expression of E- and P-cadherin in gastric carcinoma cell lines. Virchows Arch 1994; 425: pp. 139-144
- 405. Rosen E.M., et al: Scatter factor modulates the metastatic phenotype of the EMT6 mouse mammary tumor. Int J Cancer 1994; 57: pp. 706-714
- 406. Rosen E.M., et al: Smooth muscle-derived factor stimulates mobility of human tumor cells. Invasion Metastasis 1990; 10: pp. 49-64
- 407. Taniguchi T., et al: Increase in the circulating level of hepatocyte growth factor in gastric cancer patients. Br J Cancer 1997; 75: pp. 673-677
- 408. Kataoka H., et al: Activation of hepatocyte growth factor/scatter factor in colorectal carcinoma. Cancer Res 2000; 60: pp. 6148-6159
- 409. Kaji M., et al: Participation of c-met in the progression of human gastric cancers: anti-c-met oligonucleotides inhibit proliferation or invasiveness of gastric cancer cells. Cancer Gene Ther 1996; 3: pp. 393-404
- 410. Jiang W.G., et al: Regulation of spreading and growth of colon cancer cells by hepatocyte growth factor. Clin Exp Metastasis 1993; 11: pp. 235-242
- 411. Jiang W.G., et al: Inhibition of hepatocyte growth factor-induced motility and in vitro invasion of human colon cancer cells by gamma-linolenic acid. Br J Cancer 1995; 71: pp. 744-752
- 412. Iwazawa T., et al: Primary human fibroblasts induce diverse tumor invasiveness: involvement of HGF as an important paracrine factor. Jpn J Cancer Res 1996; 87: pp. 1134-1142
- 413. Hiscox S.E., et al: Expression of the HGF/SF receptor, c-met, and its ligand in human colorectal cancers. Cancer Invest 1997; 15: pp. 513-521
- 414. Fujita S., and Sugano K.: Expression of c-met proto-oncogene in primary colorectal cancer and liver metastases. Jpn J Clin Oncol 1997; 27: pp. 378-383
- 415. Di Renzo M.F., et al: Overexpression and amplification of the met/HGF receptor gene during the progression of colorectal cancer. Clin Cancer Res 1995; 1: pp. 147-154
- 416. Jiang W.G.: E-cadherin and its associated protein catenins, cancer invasion and metastasis. Br J Surg 1996; 83: pp. 437-446
- 417. Oliveira M.J., et al: [object Object]. J Biol Chem 2006; 281: pp. 34888-34896
- 418. Churin Y., et al: [object Object]. J Cell Biol 2003; 161: pp. 249-255
- 419. Bertaux-Skeirik N., et al: CD44 plays a functional role in . PLoS Pathog 2015; 11: pp. e1004663
- 420. Takaishi S., et al: Identification of gastric cancer stem cells using the cell surface marker CD44. Stem Cells 2009; 27: pp. 1006-1020
- 421. Yao Y., et al: Scatter factor protein levels in human breast cancers: clinicopathological and biological correlations. Am J Pathol 1996; 149: pp. 1707-1717
- 422. Yamashita J., et al: Immunoreactive hepatocyte growth factor is a strong and independent predictor of recurrence and survival in human breast cancer. Cancer Res 1994; 54: pp. 1630-1633
- 423. Ueda T., et al: Significant elevation of serum human hepatocyte growth factor levels in patients with acute pancreatitis. Pancreas 1996; 12: pp. 76-83
- 424. Uchiyama A., et al: Interleukin 4 inhibits hepatocyte growth factor- induced invasion and migration of colon carcinomas. J Cell Biochem 1996; 62: pp. 443-453
- 425. Scotlandi K., et al: Expression of Met/hepatocyte growth factor receptor gene and malignant behavior of musculoskeletal tumors. Am J Pathol 1996; 149: pp. 1209-1219
- 426. Pisters L.L., et al: c-met proto-oncogene expression in benign and malignant human prostate tissues. J Urol 1995; 154: pp. 293-298
- 427. Ichimura E., et al: Expression of c-met/HGF receptor in human non-small cell lung carcinomas in vitro and in vivo and its prognostic significance. Jpn J Cancer Res 1996; 87: pp. 1063-1069
- 428. Humphrey P.A., et al: Hepatocyte growth factor and its receptor (c-MET) in prostatic carcinoma. Am J Pathol 1995; 147: pp. 386-396
- 429. Date K., et al: HGF/NK4 is a specific antagonist for pleiotrophic actions of hepatocyte growth factor. FEBS Lett 1997; 420: pp. 1-6
- 430. Danopoulos S., et al: Fibroblast growth factors in the gastrointestinal tract: twists and turns. Dev Dyn 2017; 246: pp. 344-352
- 431. Ornitz D.M., and Itoh N.: The fibroblast growth factor signaling pathway. Wiley Interdiscip Rev Dev Biol 2015; 4: pp. 215-266
- 432. Degirolamo C., Sabba C., and Moschetta A.: Therapeutic potential of the endocrine fibroblast growth factors FGF19, FGF21 and FGF23. Nat Rev Drug Discov 2016; 15: pp. 51-69
- 433. Kuro-o M.: Endocrine FGFs and Klothos: emerging concepts. Trends Endocrinol Metab 2008; 19: pp. 239-245
- 434. Potthoff M.J., Kliewer S.A., and Mangelsdorf D.J.: Endocrine fibroblast growth factors 15/19 and 21: from feast to famine. Genes Dev 2012; 26: pp. 312-324
- 435. Gavalda-Navarro A., et al: Fibroblast growth factor 21 in breast milk controls neonatal intestine function. Sci Rep 2015; 5: pp. 13717
- 436. Saito T., and Fukumoto S.: Fibroblast growth factor 23 (FGF23) and disorders of phosphate metabolism. Int J Pediatr Endocrinol 2009; 2009: pp. 496514
- 437. Al Alam D., et al: Fibroblast growth factor 10 alters the balance between goblet and Paneth cells in the adult mouse small intestine. Am J Physiol Gastrointest Liver Physiol 2015; 308: pp. G678-G690
- 438. Al Alam D., et al: FGF9-Pitx2-FGF10 signaling controls cecal formation in mice. Dev Biol 2012; 369: pp. 340-348
- 439. Houchen C.W., et al: FGF-2 enhances intestinal stem cell survival and its expression is induced after radiation injury. Am J Physiol 1999; 276: pp. G249-G258
- 440. Zhang X., et al: Reciprocal epithelial-mesenchymal FGF signaling is required for cecal development. Development 2006; 133: pp. 173-180
- 441. Vidrich A., et al: Fibroblast growth factor receptor-3 is expressed in undifferentiated intestinal epithelial cells during murine crypt morphogenesis. Dev Dyn 2004; 230: pp. 114-123
- 442. Stark K.L., McMahon J.A., and McMahon A.P.: FGFR-4, a new member of the fibroblast growth factor receptor family, expressed in the definitive endoderm and skeletal muscle lineages of the mouse. Development 1991; 113: pp. 641-651
- 443. La Rosa S., et al: Immunohistochemical localization of acidic fibroblast growth factor in normal human enterochromaffin cells and related gastrointestinal tumours. Virchows Arch 1997; 430: pp. 117-124
- 444. Capetandes A., et al: Acidic fibroblast growth factor is present in the enteric nervous system of the large intestine. J Histochem Cytochem 2000; 48: pp. 407-414
- 445. Nakayama F., et al: Post treatment with an FGF chimeric growth factor enhances epithelial cell proliferation to improve recovery from radiation-induced intestinal damage. Int J Radiat Oncol Biol Phys 2010; 78: pp. 860-867
- 446. Fu X.B., et al: Enhanced anti-apoptosis and gut epithelium protection function of acidic fibroblast growth factor after cancelling of its mitogenic activity. World J Gastroenterol 2004; 10: pp. 3590-3596
- 447. Li H.H., et al: Non-mitogenic acidic fibroblast growth factor reduces intestinal dysfunction induced by ischemia and reperfusion injury in rats. J Gastroenterol Hepatol 2007; 22: pp. 363-370
- 448. Liu L.M., Russell S.M., and Nicoll C.S.: Analysis of the role of basic fibroblast growth factor in growth and differentiation of transplanted fetal rat paws and intestines. Endocrinology 1990; 126: pp. 1764-1770
- 449. Gonzalez A.M., et al: Distribution of fibroblast growth factor (FGF)-2 and FGF receptor-1 messenger RNA expression and protein presence in the mid-trimester human fetus. Pediatr Res 1996; 39: pp. 375-385
- 450. Song X., et al: Growth factor FGF2 cooperates with interleukin-17 to repair intestinal epithelial damage. Immunity 2015; 43: pp. 488-501
- 451. Dignass A.U., Tsunekawa S., and Podolsky D.K.: Fibroblast growth factors modulate intestinal epithelial cell growth and migration. Gastroenterology 1994; 106: pp. 1254-1262
- 452. Lotz S., et al: Sustained levels of FGF2 maintain undifferentiated stem cell cultures with biweekly feeding. PLoS One 2013; 8: pp. e56289
- 453. Yang Y., et al: Heightened potency of human pluripotent stem cell lines created by transient BMP4 exposure. Proc Natl Acad Sci U S A 2015; 112: pp. E2337-E2346
- 454. Fu X.B., et al: Rapid mitogen-activated protein kinase by basic fibroblast growth factor in rat intestine after ischemia/reperfusion injury. World J Gastroenterol 2003; 9: pp. 1312-1317
- 455. Chen W., et al: Exogenous acid fibroblast growth factor inhibits ischemia-reperfusion-induced damage in intestinal epithelium via regulating P53 and P21WAF-1 expression. World J Gastroenterol 2005; 11: pp. 6981-6987
- 456. Spence J.R., et al: Directed differentiation of human pluripotent stem cells into intestinal tissue in vitro. Nature 2011; 470: pp. 105-109
- 457. Ameri J., et al: FGF2 specifies hESC-derived definitive endoderm into foregut/midgut cell lineages in a concentration-dependent manner. Stem Cells 2010; 28: pp. 45-56
- 458. Sasaki H., et al: HST-1/FGF-4 plays a critical role in crypt cell survival and facilitates epithelial cell restitution and proliferation. Oncogene 2004; 23: pp. 3681-3688
- 459. Zeeh J.M., et al: Keratinocyte growth factor ameliorates mucosal injury in an experimental model of colitis in rats. Gastroenterology 1996; 110: pp. 1077-1083
- 460. Iwakiri D., and Podolsky D.K.: Keratinocyte growth factor promotes goblet cell differentiation through regulation of goblet cell silencer inhibitor. Gastroenterology 2001; 120: pp. 1372-1380
- 461. Cai Y.J., et al: Up-regulation of intestinal epithelial cell derived IL-7 expression by keratinocyte growth factor through STAT1/IRF-1, IRF-2 pathway. PLoS One 2013; 8: pp. e58647
- 462. Visco V., et al: Human colon fibroblasts induce differentiation and proliferation of intestinal epithelial cells through the direct paracrine action of keratinocyte growth factor. J Cell Physiol 2009; 220: pp. 204-213
- 463. Egger B., et al: Keratinocyte growth factor ameliorates dextran sodium sulfate colitis in mice. Dig Dis Sci 1999; 44: pp. 836-844
- 464. Haxhija E.Q., et al: Intestinal epithelial cell proliferation is dependent on the site of massive small bowel resection. Pediatr Surg Int 2007; 23: pp. 379-390
- 465. Johnson W.F., et al: Keratinocyte growth factor enhances early gut adaptation in a rat model of short bowel syndrome. Vet Surg 2000; 29: pp. 17-27
- 466. Washizawa N., et al: Comparative effects of glucagon-like peptide-2 (GLP-2), growth hormone (GH), and keratinocyte growth factor (KGF) on markers of gut adaptation after massive small bowel resection in rats. JPEN J Parenter Enteral Nutr 2004; 28: pp. 399-409
- 467. Cai Y.J., et al: Keratinocyte growth factor up-regulates Interleukin-7 expression following intestinal ischemia/reperfusion in vitro and in vivo. Int J Clin Exp Pathol 2012; 5: pp. 569-580
- 468. Nyeng P., et al: Fibroblast growth factor 10 represses premature cell differentiation during establishment of the intestinal progenitor niche. Dev Biol 2011; 349: pp. 20-34
- 469. Tai C.C., et al: Induction of fibroblast growth factor 10 (FGF10) in the ileal crypt epithelium after massive small bowel resection suggests a role for FGF10 in gut adaptation. Dev Dyn 2009; 238: pp. 294-301
- 470. Miceli R., et al: Efficacy of keratinocyte growth factor-2 in dextran sulfate sodium-induced murine colitis. J Pharmacol Exp Ther 1999; 290: pp. 464-471
- 471. Greenwood-Van Meerveld B., Venkova K., and Connolly K.: Efficacy of repifermin (keratinocyte growth factor-2) against abnormalities in gastrointestinal mucosal transport in a murine model of colitis. J Pharm Pharmacol 2003; 55: pp. 67-75
- 472. Sandborn W.J., et al: Repifermin (keratinocyte growth factor-2) for the treatment of active ulcerative colitis: a randomized, double-blind, placebo-controlled, dose-escalation trial. Aliment Pharmacol Ther 2003; 17: pp. 1355-1364
- 473. Hamady Z.Z., et al: Xylan-regulated delivery of human keratinocyte growth factor-2 to the inflamed colon by the human anaerobic commensal bacterium . Gut 2010; 59: pp. 461-469
- 474. Geske M.J., et al: Fgf9 signaling regulates small intestinal elongation and mesenchymal development. Development 2008; 135: pp. 2959-2968
- 475. Vidrich A., et al: Fibroblast growth factor receptor-3 regulates Paneth cell lineage allocation and accrual of epithelial stem cells during murine intestinal development. Am J Physiol Gastrointest Liver Physiol 2009; 297: pp. G168-G178
- 476. Hu M.C., et al: FGF-18, a novel member of the fibroblast growth factor family, stimulates hepatic and intestinal proliferation. Mol Cell Biol 1998; 18: pp. 6063-6074
- 477. King S.L., Mohiuddin J.J., and Dekaney C.M.: Paneth cells expand from newly created and preexisting cells during repair after doxorubicin- induced damage. Am J Physiol Gastrointest Liver Physiol 2013; 305: pp. G151-G162
- 478. Kanai M., Rosenberg I., and Podolsky D.K.: Cytokine regulation of fibroblast growth factor receptor 3 IIIb in intestinal epithelial cells. Am J Physiol 1997; 272: pp. G885-G893
- 479. Tanner Y., and Grose R.P.: Dysregulated FGF signalling in neoplastic disorders. Semin Cell Dev Biol 2016; 53: pp. 126-135
- 480. Nakazawa K., Yashiro M., and Hirakawa K.: Keratinocyte growth factor produced by gastric fibroblasts specifically stimulates proliferation of cancer cells from scirrhous gastric carcinoma. Cancer Res 2003; 63: pp. 8848-8852
- 481. Bai Y.P., et al: FGF-1/-3/FGFR4 signaling in cancer-associated fibroblasts promotes tumor progression in colon cancer through Erk and MMP-7. Cancer Sci 2015; 106: pp. 1278-1287
- 482. Kirikoshi H., et al: Molecular cloning and characterization of human FGF-20 on chromosome 8p21.3-p22. Biochem Biophys Res Commun 2000; 274: pp. 337-343
- 483. Sonvilla G., et al: FGF18 in colorectal tumour cells: autocrine and paracrine effects. Carcinogenesis 2008; 29: pp. 15-24
- 484. Ribieras S., Tomasetto C., and Rio M.C.: The pS2/TFF1 trefoil factor, from basic research to clinical applications. Biochim Biophys Acta 1998; 1378: pp. F61-F77
- 485. Poulsom R., and Wright N.A.: Trefoil peptides: a newly recognized family of epithelial mucin-associated molecules. Am J Physiol 1993; 265: pp. G205-G213
- 486. Hoffmann W., and Jagla W.: Cell type specific expression of secretory TFF peptides: colocalization with mucins and synthesis in the brain. Int Rev Cytol 2002; 213: pp. 147-181
- 487. Hoffmann W., and Hauser F.: The P-domain or trefoil motif: a role in renewal and pathology of mucous epithelia? Trends Biochem Sci 1993; 18: pp. 239-243
- 488. Thim L.: Trefoil peptides: from structure to function. Cell Mol Life Sci 1997; 53: pp. 888-903
- 489. Taupin D., and Podolsky D.K.: Trefoil factors: initiators of mucosal healing. Nat Rev Mol Cell Biol 2003; 4: pp. 721-732
- 490. Sands B.E., and Podolsky D.K.: The trefoil peptide family. Annu Rev Physiol 1996; 58: pp. 253-273
- 491. Podolsky D.K.: Mechanisms of regulatory peptide action in the gastrointestinal tract: trefoil peptides. J Gastroenterol 2000; 35: pp. 69-74
- 492. Katoh M.: Trefoil factors and human gastric cancer. Int J Mol Med 2003; 12: pp. 3-9
- 493. Bossenmeyer-Pourie C., et al: The trefoil factor 1 participates in gastrointestinal cell differentiation by delaying G1-S phase transition and reducing apoptosis. J Cell Biol 2002; 157: pp. 761-770
- 494. Wright N.A., et al: Trefoil peptide gene expression in gastrointestinal epithelial cells in inflammatory bowel disease. Gastroenterology 1993; 104: pp. 12-20
- 495. Cook G.A., et al: The trefoil peptides TFF2 and TFF3 are expressed in rat lymphoid tissues and participate in the immune response. FEBS Lett 1999; 456: pp. 155-159
- 496. Newton J.L., et al: The human trefoil peptide, TFF1, is present in different molecular forms that are intimately associated with mucus in normal stomach. Gut 2000; 46: pp. 312-320
- 497. Jeffrey G.P., et al: Spasmolytic polypeptide: a trefoil peptide secreted by rat gastric mucous cells. Gastroenterology 1994; 106: pp. 336-345
- 498. Masiakowski P., et al: Cloning of cDNA sequences of hormone-regulated genes from the MCF-7 human breast cancer cell line. Nucleic Acids Res 1982; 10: pp. 7895-7903
- 499. Jorgensen K.D., et al: Pancreatic spasmolytic polypeptide (PSP): III. Pharmacology of a new porcine pancreatic polypeptide with spasmolytic and gastric acid secretion inhibitory effects. Regul Pept 1982; 3: pp. 231-243
- 500. Suemori S., Lynch-Devaney K., and Podolsky D.K.: Identification and characterization of rat intestinal trefoil factor: tissue- and cell-specific member of the trefoil protein family. Proc Natl Acad Sci U S A 1991; 88: pp. 11017-11021
- 501. Hirota M., Furukawa Y., and Hayashi K.: Expression of pS2 gene in human breast cancer cell line MCF-7 is controlled by retinoic acid. Biochem Int 1992; 26: pp. 1073-1078
- 502. Cavailles V., Garcia M., and Rochefort H.: Regulation of cathepsin-D and pS2 gene expression by growth factors in MCF7 human breast cancer cells. Mol Endocrinol 1989; 3: pp. 552-558
- 503. Peterson A.J., et al: [object Object]. Gastroenterology 2010; 139: pp. 2005-2017
- 504. Tran C.P., et al: Short-chain fatty acids inhibit intestinal trefoil factor gene expression in colon cancer cells. Am J Physiol 1998; 275: pp. G85-G94
- 505. Ribieras S., et al: Mouse Trefoil factor genes: genomic organization, sequences and methylation analyses. Gene 2001; 266: pp. 67-75
- 506. Ogata H., and Podolsky D.K.: Trefoil peptide expression and secretion is regulated by neuropeptides and acetylcholine. Am J Physiol 1997; 273: pp. G348-G354
- 507. Furuta G.T., et al: Hypoxia-inducible factor 1-dependent induction of intestinal trefoil factor protects barrier function during hypoxia. J Exp Med 2001; 193: pp. 1027-1034
- 508. Van der Sluis M., et al: Muc2-deficient mice spontaneously develop colitis, indicating that MUC2 is critical for colonic protection. Gastroenterology 2006; 131: pp. 117-129
- 509. Gum J.R., Hicks J.W., and Kim Y.S.: Identification and characterization of the MUC2 (human intestinal mucin) gene 5′-flanking region: promoter activity in cultured cells. Biochem J 1997; 325: pp. 259-267
- 510. Tomasetto C., et al: pS2/TFF1 interacts directly with the VWFC cysteine-rich domains of mucins. Gastroenterology 2000; 118: pp. 70-80
- 511. Xiao P., et al: Trefoil factors: gastrointestinal-specific proteins associated with gastric cancer. Clin Chim Acta 2015; 450: pp. 127-134
- 512. Buda A., Jepson M.A., and Pignatelli M.: Regulatory function of trefoil peptides (TFF) on intestinal cell junctional complexes. Cell Commun Adhes 2012; 19: pp. 63-68
- 513. Aihara E., Engevik K.A., and Montrose M.H.: Trefoil factor peptides and gastrointestinal function. Annu Rev Physiol 2017; 79: pp. 357-380
- 514. Aamann L., Vestergaard E.M., and Gronbaek H.: Trefoil factors in inflammatory bowel disease. World J Gastroenterol 2014; 20: pp. 3223-3230
- 526. Ruiz I., and Altaba A.: Gli proteins and Hedgehog signaling: development and cancer. Trends Genet 1999; 15: pp. 418-425
- 527. Xiao C., et al: Loss of parietal cell expression of Sonic Hedgehog induces hypergastrinemia and hyperproliferation of surface mucous cells. Gastroenterology 2010; 138: pp. 550-561
- 528. van den Brink G.R., et al: Sonic Hedgehog regulates gastric gland morphogenesis in man and mouse. Gastroenterology 2001; 121: pp. 317-328
- 529. Zacharias W.J., et al: Hedgehog signaling controls homeostasis of adult intestinal smooth muscle. Dev Biol 2011; 355: pp. 152-162
- 530. Kolterud A., et al: Paracrine Hedgehog signaling in stomach and intestine: new roles for Hedgehog in gastrointestinal patterning. Gastroenterology 2009; 137: pp. 618-628
- 531. Schumacher M.A., et al: Gastric Sonic Hedgehog acts as a macrophage chemoattractant during the immune response to . Gastroenterology 2012; 142: pp. 1150-1159
- 532. Engevik A.C., et al: The acid-secreting parietal cell as an endocrine source of Sonic Hedgehog during gastric repair. Endocrinology 2013; 154: pp. 4627-4639
- 533. El-Zaatari M., et al: Plasma Shh levels reduced in pancreatic cancer patients. Pancreas 2012; 41: pp. 1019-1028
- 534. Ding L., et al: Schlafen 4-expressing myeloid-derived suppressor cells are induced during murine gastric metaplasia. J Clin Invest 2016; 126: pp. 2867-2880
- 535. Braune J., et al: Hedgehog signalling in myeloid cells impacts on body weight, adipose tissue inflammation and glucose metabolism. Diabetologia 2017; 60: pp. 889-899
- 536. Zavros Y., et al: Sonic Hedgehog is associated with H . Am J Physiol Gastrointest Liver Physiol 2008; 295: pp. G99-111
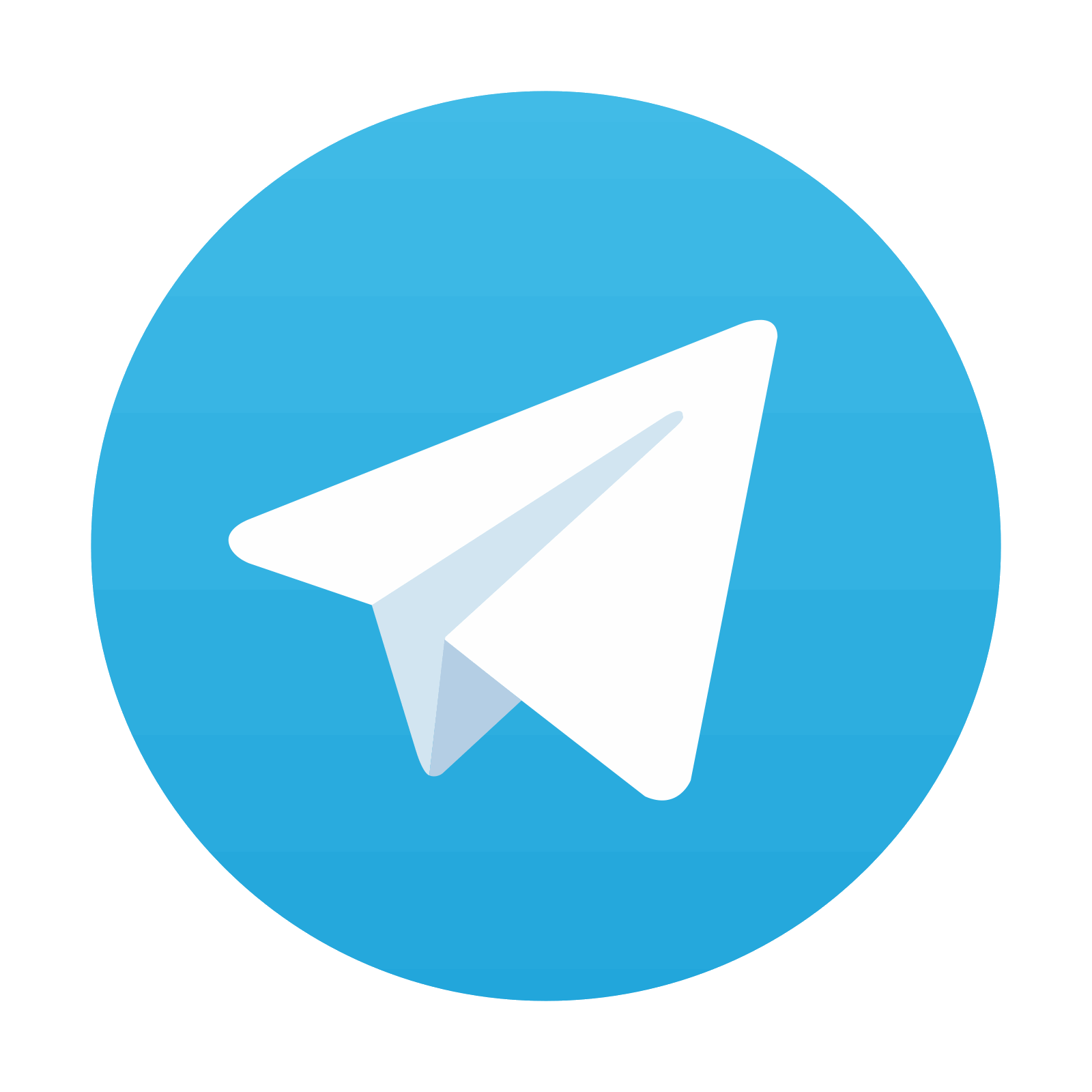
Stay updated, free articles. Join our Telegram channel
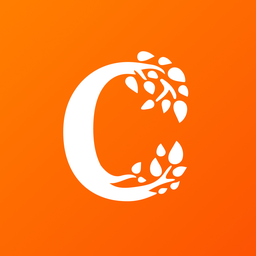
Full access? Get Clinical Tree
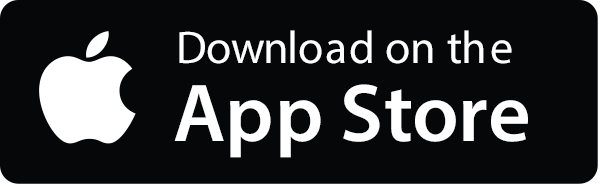
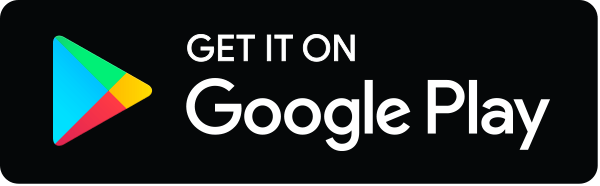