Refluxate toxicity
Gastric acid secretion
Duodenogastric reflux
Intrinsic gastric volume and pressure
Gastric compliance
Gastric emptying
Gastric acid volume secretion
Extrinsic pressure on gastric contents
Weight (obesity)
Somatic motor tone (spasticity)
Somatic and crural episodic contractions (cough, wheeze, others)
Gastroesophageal barrier
Lower esophageal sphincter tone
Gastric fundic sensory thresholds (for transient lower esophageal sphincter relaxations)
Crural diaphragm location (relative to sphincter location) and function
Esophageal defenses
Salivary secretion
Peristaltic motor function
Esophageal cytoprotection
The first studies to infer a genetic component to GERD were case reports describing familial clusters of radiologically confirmed hiatal hernia (reviewed in [3]). In the largest and most detailed of these studies, Carre and colleagues described a kindred of 38 family members across five generations, all of whom were interviewed and subjected to a barium meal. Among this pedigree were 20 individuals with both symptoms of gastroesophageal reflux, and radiologic evidence of hiatal hernia. An autosomal dominant mode of inheritance was suggested [4].
Although familial clusters of hiatal hernia are established, most cases of hiatal hernia are sporadic and most pediatric GERD is not associated with hiatal hernia. Therefore hiatal hernia probably accounts for a small minority of cases of hereditary GERD. Other studies support a familial predisposition to GERD even in the absence of a hiatal hernia. In case control studies, GERD symptoms in relatives of patients with Barrett’s metaplasia, and esophageal adenocarcinoma were more prevalent than in spouse controls. A similar increased prevalence among relatives of patients with uncomplicated esophagitis was not observed in this study, suggesting that only severe GERD is heritable [5]. However, another investigation of patients with abnormal pH studies revealed that relatives of patients with increased esophageal acid exposure were more likely to experience frequent reflux symptoms, even in the absence of GERD complications [6].
Familial clustering of GERD can also arise from shared environmental risk factors rather than genetic factors. In twin studies , conditions that bear a large genetic predisposition are more likely concordant in monozygotic twins than dizygotic twins. Two large-scale studies have investigated the concordance of GERD in mono- and dizygotic twins. In one of these studies, twins belonging to the national Swedish Twin Registry were queried for GERD using a questionnaire. In almost 3100 twin pairs with GERD, aged 55 years or greater, the concordance was significantly higher among monozygotic twins compared to dizygotic twins, suggesting that heritability accounts for approximately one-third of the susceptibility to GERD [7]. A similar study from a twin registry in the United Kingdom corroborated the Swedish study, finding concordance rates of 42 % for monozygotic twins versus 26 % for dizygotic twins, and concluding that 43 % of the predilection to GERD is genetically influenced [8].
In an attempt to detect specific genetic loci associated with GERD, Hu and colleagues performed a genetic linkage analysis of five families in which multiple family members were afflicted with severe pediatric GERD. They found a 9-centiMorgan locus on the long arm of chromosome 13 (13q14; termed GERD1) that segregated with the severe pediatric GERD phenotype in their cohort [9]. A candidate gene in this region is the 5-hydroxytryptamine receptor 2A. However, no coding sequence mutations were identified [9, 10]. The authors proposed that mutations in regulatory or other noncoding regions, null alleles , segmental deletions and duplications, and epigenetic effects in the 13q14 region could still account for the pediatric GERD phenotype in these families [10]. In support of this, a case report describes a dysmorphic infant with severe GERD who possesses a 12.8 megabase deletion spanning the GERD1 locus, implicating GERD1 haploinsufficiency in the patient’s symptoms [11]. However, independent linkage analyses in different cohorts failed to confirm the association of the 13q14 locus with GERD [2, 12] underscoring the genetic heterogeneity of GERD.
The subjective sensation of GER has been attributed to the exposure of esophageal mucosa to the acidic gastric refluxate causing a mucosal inflammatory response which, in turn, activates afferent sensory nerve pathways. As such, variations in genes that participate in inflammation, wound healing, and sensory neuromodulation could theoretically contribute to the experience of gastroesophageal reflux . With this in mind, three recent studies have focused on genes in these pathways. A study by Chourasia et al. evaluated the relationship of polymorphisms in genes encoding the Interleukin 1B (IL-1B) and the interleukin one receptor antagonist (IL-1RN) to GERD in patients referred to a tertiary center [13]. A single nucleotide polymorphism in the promoter region of the IL-1B gene predisposes to increased concentrations of IL-1beta, a potent pro-inflammatory cytokine. In contrast, a polymorphism consisting of two intronic tandem repeats (as opposed to 3–5 repeats) within the IL-1RN gene tends to decrease IL-1beta levels and has an anti-inflammatory effect. Hypothesizing that increased gastric inflammation destroys proton-excreting parietal cells, thus lowering esophageal acid exposure, investigators characterized the IL-1 genotypes of 144 patients with confirmed GERD and 368 healthy controls. They found that subjects with genotypes and haplotypes combining to decrease IL-1beta levels had predictably lower gastric mucosal IL-1beta expression, and generally had a higher risk of GERD. These genotypes and haplotypes were also more prevalent in the GERD population [13].
In another genome-wide association study, Asling and colleagues collected 36 GERD-affected families and mapped familial GERD to a 35 megabase pair region on chromosome 2q24-q33, confirming this association in a separate cohort. Biopsies from those subjects with abnormal endoscopic or pHmetric findings, or those who had undergone fundoplication, were then subjected to gene expression analysis and the COL3A1 gene (collagen type III alpha I), residing within this locus, was found to be differentially expressed in subjects with GERD compared to controls. As collagen type III contributes to tissue strength, flexibility, and wound response, the authors proposed a mechanism whereby altered collagen type III expression in the esophagus results in a predisposition to esophageal damage in patients with gastroesophageal reflux. Although they immunohistochemically demonstrated increased expression of the collagen III protein in esophageal tissue biopsies, they did not investigate whether this was a cause or a consequence of gastroesophageal reflux. Also, sequencing of the COL3A1 gene in 48 subjects did not identify any causative mutations. The authors propose that disease-causing mutations reside in regulatory regions [12].
G-proteins are second messengers involved in the neurotransmission of gastroesophageal sensation . The C825T substitution polymorphism within the gene encoding the G-protein β3 subunit results in enhanced G-protein activation and signal transduction [14], and is associated with functional dyspepsia [15]. On this basis, De Vries and coworkers explored the relationship of the C825T allele with GERD in 363 subjects with either pathologic esophageal acid exposure or a positive symptoms association score for heartburn or regurgitation. Compared to healthy controls, individuals with GERD were more likely to be heterozygous for the C825T. The likelihood of being heterozygous for C825T was highest (adjusted odds ratio 1.5; 95 % CI 1.06–2.13) among patients with a positive symptom association score, but no correlation was observed among those with pathologic acid exposure. This suggests that enhanced perception of physiologic acid exposure, and not pathologic acid exposure, underlies the association of C825T heterozygosity and GERD [16].
More recently, larger databases of better characterized subjects have enabled more statistically powerful genome-wide association studies quantifying the genetic contributions to GERD. Ek et al. analyzed a Swedish cohort of 994 subjects and over 600,000 Single Nucleotide Polymorphisms (SNPs), and did not identify a genetic contribution to the risk of GERD, probably because this study was still limited by an inadequate sample size [17]. More recently, however, interrogation of the Barrett’s and Esophageal Adenocarcinoma Consortium (BEACON) and the 23 and ME discovery cohorts, which contain detailed information on heartburn and regurgitation symptoms from almost 10,000 GERD cases, demonstrated for the first time that GERD has a polygenic background, but that no individual specific SNPs conferred GERD susceptibility [18]. However, using a specific set of SNPs, they were able to predict the risk of GERD in an independent target cohort and there was an estimated SNP heritability of 7 % (95 % CI 3–11 %). It should be noted that these were adult studies and the genetic underpinnings of GERD in children may differ.
Each of the above studies propose different heritable host factors in the pathophysiology of GERD, increasing the likelihood that still other host factors exist. Furthermore, replication and validation of existing studies is required, highlighting the great need for further investigation in this area.
Triple A Syndrome
Triple A syndrome (AAAS) is a condition characterized by alacrima, adrenocorticotrophic hormone-resistant adrenal insufficiency, and achalasia [19]. Although its name connotes a triad, the syndrome is phenotypically heterogeneous : fewer than three features may be present and additional features not originally identified in the initial report by Allgrove , including progressive autonomic, central, and peripheral nervous system deficits, are associated with the syndrome [20]. The etiology of achalasia in AAAS appears to be distinct from other forms of achalasia [21]; in contrast to idiopathic achalasia, which does not segregate in families, AAAS is a genetic disorder. Although it is a rare condition and epidemiologic data are scant, symptoms of swallowing difficulty and achalasia in AAAS usually manifests by the end of the first decade of life and can begin in infancy [22, 23]—in contrast to idiopathic achalasia, where very small minority of patients manifest symptoms before 10 years of age [24]. However, there is also a late-onset form of AAAS [25]. The diagnosis of achalasia in AAAS relies on the same manometric, radiographic, and endoscopic criteria as for idiopathic achalasia, and the treatment is similar. However, AAAS is thought to follow a more severe course [26].
Frameshift, point, or missense mutations in the gene AAAS, located at 12q13, account for the majority of cases of AAAS [20, 27, 28]. Consanguinity is often present in kindreds of AAAS and the condition mostly segregates in an autosomal recessive pattern, but compound heterozygous mutations in AAAS have resulted in AAAS, sometimes with pleiotropic effects. In total, greater than 70 homozygous or compound heterozygous mutations have been identified [29]. The penetrance of AAAS with bi-allelic mutations in AAAS approaches 100 %, though expressivity is variable, possibly due to abovementioned allelic variation or the existence of as yet unidentified modifier genes. Conversely, others have reported a very tight genotype–phenotype correlation [30].
AAAS encodes the ALADIN protein (an acronym for alacrima, achalasia, adrenal insufficiency and neurological disorder) [31, 32]. ALADIN is part of the nuclear pore complex , a large, multiprotein complex spanning the nuclear envelope and forming a selective channel between the cytoplasm and the nucleus [33, 34]. ALADIN is the first nuclear pore protein linked to a human heritable disease. In most cases of AAAS, ALADIN is truncated, resulting in its exclusion from nuclear pore complex and in ectopic cytoplasmic accumulation. This may be due to an inability of the mutant ALADIN protein to anchor to proteins required for nuclear pore complex assembly [35] or to the deletion of a critical nuclear localization signals in the AAAS transcript [36]. Although the nucleus and the nuclear pore complex remain morphologically and structurally intact without ALADIN, the nuclear import of selected proteins is interrupted. Proteins involved in DNA repair and the attenuation of oxidative stress fail to localize to the nucleus when ALADIN is absent from the nuclear pore complex [37, 38]. On this basis, it is proposed that defective ALADIN renders cells susceptible to oxidative DNA damage and cell death in a tissue-specific manner [37]. In support of this, fibroblast cultures derived from patients with AAAS possess a higher basal level of reactive oxygen species, a heightened response to oxidative stress and a predilection for premature stress-induced senescence [39]. Similar findings were observed in an AAAS knockdown cell line model [40].
In addition to its role in redox homeostasis, ALADIN may also be required for proper mitotic spindle assembly, as artificially depleting human cells of ALADIN in vitro slows spindle assembly and chromosome alignment, by disrupting the recruitment of other spindle proteins (Aurora A) [41]. Indeed, the observation that mitotic errors are common in triple A patient fibroblasts supports the possibility that mitotic defects also play a role in AAAS.
Given the variety and abundance of macromolecules that depend on a competent nuclear pore complex and a functional mitotic spindle, it is probable that additional genes and proteins will one day be identified that will further elucidate how the ALADIN defect translates into the observed phenotypes of AAAS.
Hirschsprung Disease
Hirschsprung disease (HSCR) is the developmental abnormality resulting from the failure of vagal neural crest cells to complete colonization of the developing intestine between the 5th and 12th week of gestation [42, 43]. A minority of HSCR (30 %) is syndromic (associated with other congenital anomalies) with several monogenic syndromes recognized and chromosomal abnormalities found in 12 % of cases. The overt abnormality is the absence of intramural ganglion cells in the myenteric and submucosal plexuses of the rectum and a variable length of contiguous bowel. HSCR is classified by length of aganglionosis into short-segment (70 % of cases), long-segment (20 % of cases) with aganglionosis rostral to the splenic flexure, and with total colonic (10 % of cases) aganglionosis.
HSCR is a sex-modified, multifactorial genetic disease [44]. Males are affected more frequently than females with the gender bias being higher for short-segment (4.2–4.4) than for long-segment disease (1.2–1.9) [44]. HSCR is associated with other congenital anomalies in 30 % of cases including recognized syndromes (18 %) and chromosome abnormalities (12 %) [45]. Associated anomalies include pigmentary, sensorineural, craniofacial, urogenital and gastrointestinal (atresia/stenosis of the colon, rectum, anal canal), cleft lip/palate, polydactyly, and cardiac defects. HSCR is a mandatory feature of 11 syndromes , including Shah-Waardenburg, Goldberg–Shprintzen, Mowat–Wilson, and Congenital Central Hypoventilation (CCHS) syndromes, and a non-obligate finding in 30 others, such as Bardet–Biedl, Kaufman–McKusick, and Smith–Lemli–Opitz syndromes. Trisomy 21 (2–10 % of cases) and deletion/duplication of chromosome 17q21-23 are common, as are large deletions of RET, EDNRB, and ZFHX1B [45]. The complex genetics of HSCR is reflected in its varied phenotype, recurrence risk, and penetrance in families, features that modulate its presentation and clinical outcome.
Genetic studies have identified rare high-penetrance mutations in 13 genes (RET, GDNF, NRTN, SOX10, EDNRB, EDN3, ECE1, ZFHX1B, PHOX2B, KBP, TCF4, L1CAM, and IKBKAP) predominant in syndromic HSCR [46]. Recent exome sequencing studies have identified several new genes [47, 48]. Approximately 30 % of patients have one of these rare mutations [49].
In contrast, three common noncoding and low-penetrance variants at RET (rs2435357, rs2506030, and rs7069590) [50, 51] and at least one each at NRG1 (rs4541858) [52] and SEMA3C/D (rs11766001) [53] contribute to pathogenesis and length of aganglionosis in isolated HSCR. All three noncoding variants of RET increase disease risk through significant reduction of binding of the transcription factors SOX10, RARB, and GATA2 to RET enhancers, leading to reduced RET expression in the developing gut. Kapoor et al. analyzed these variants in 997 samples from 376 HSCR families of European ancestry and reported that individuals with one or fewer risk alleles (∼50 % of the population) have an estimated risk of ∼1/20,000 live births and those with two risk alleles (27.3 % of the population) have a background risk of HSCR at ∼15/100,000 live births. However, individuals with three or more risk alleles (23.3 % of the population) have an increased HSCR risk varying from 1.6- to 9.5-fold. Those with five or six risk alleles have an estimated risk of ∼1/800 live births. Thus there is a 32-fold range in risk based on these alleles, with an odds ratio varying from 0.3 to 9.5 between those with 0 versus 5 or 6 alleles. The variations are even more marked when individuals are classified by gender [49]. Overall, HSCR can be caused by the segregation of multiple common and rare variants in at least 23 genes and 15 chromosomal loci but a RET loss-of-function allele appears to be necessary for disease expression [49].
The genes implicated in HSCR affect the migration, proliferation, survival, and/or differentiation of all enteric neural precursors, as well as the many other cell types in other organs. Consequently, the multifactorial nature of HSCR gene defects disrupts other aspects of development. The range of associated defects is wide, including obvious congenital malformation and increased risk of neoplasia in adults.
RET is a proto-oncogene that is disease causing in Multiple Endocrine Neoplasia syndrome 2 (MEN2) , congenital abnormalities of the kidney and urinary tract, and HSCR [54]. The majority of RET coding sequence mutations that cause HSCR are loss-of-function mutations that either result in early truncation or reduced function of the protein. However, gain-of-function mutations have also been identified in HSCR. These gain-of-function mutations overlap the mutations causing MEN2A and medullary thyroid carcinoma (MTC). MEN2A mutations occur in a cluster of cysteines on exon 10 or exon 11. HSCR and MEN2A occur together in some patients and families, requiring a more complex explanation for the signaling consequences of these mutations [55]. Exon 10 mutations account for all cases of HSCR associated with MEN2A, with the C620 mutation being the most common [56]. The mechanism of the dual loss-of-function and gain-of-function actions of these mutations is not fully understood. A provocative long-term follow-up study from Finnish Cancer Registry of an unselected group of 156 adults with HSCR screened for thyroid malignancy genetically and clinically found MTC or MTC-producing RET mutations in 5 % of the group (including both exon 10 and exon 13 RET mutations). They emphasized that clinical thyroid assessment did not improve the accuracy of the genetic screening and that length of aganglionosis did not correlate with the risk for thyroid malignancy. These researchers advocate for genetic screening of all individuals with HSCR [57].
Syndromic HSCR
A wide range of isolated anomalies are reported with HSCR. Cardiac defects (most commonly atrial- or ventricular-septal defects) and renal anomalies are found in ~5 % of HSCR patients and should be looked for systematically. For HSCR associated with other congenital anomalies, the prognosis is largely dependent on the severity of the other anomalies. Numerous syndromes are associated with HSCR and the recognition of these syndromes is important for disease prognosis and accurate genetic counseling. Careful evaluation by a clinician familiar with the varied associated syndromes is extremely valuable to the patients and their families. Below we discuss syndromes most commonly associated with HSCR.
HSCR occurs in syndromes with defects in other neural crest-derived tissues, termed neurocristopathies. The neural crest is a transient, multipotent, migratory cell population in the embryo that give rise to diverse tissues of the body, including melanocytes, craniofacial cartilage and bone, cells in the thymus, the cardiac outflow tract, the adrenal medulla, the autonomic nervous system, and the ENS. Congenital central hypoventilation syndrome (CCHS) is an autosomal dominant neurocristopathy characterized by an abnormal ventilatory response to hypoxia and hypercapnia due to abnormal autonomic respiratory control. The syndrome can be associated with broader dysfunction of the autonomic nervous system and with neural crest-derived tumors (5–10 % of CCHS patients develop neuroblastoma, ganglioblastoma, or ganglioneuroma). CCHS is caused by mutation in PHOX2B with a de novo heterozygous in-frame duplication leading to polyalanine expansion being the most common mutation identified. However, approximately 10 % of the parents of CCHS patients will be mosaic for the mutation and may develop late onset central hypoventilation. There is also a clear genotype–phenotype correlation with the risk for tumor development: individuals carrying the most common polyalanine expansion mutation can be reassured, while those carrying a frameshift mutation are at high risk and should be considered for regular screening [58, 59]. Overall, 20 % of individuals with CCHS have HSCR with L-HSCR or TCA being most common with a near equal male-to-female ratio [60]. However, the T allele of RET affects the penetrance of HSCR with the incidence of HSCR climbing to 60 % in CCHS patients homozygous for the T allele [61].
The combination of Waardenburg syndrome and HSCR is termed Waardenburg syndrome type 4 (WS4) or the Shah-Waardenburg syndrome. It is called by homozygous mutations of the endothelin-B signaling pathway (EDNRB or EDN3) or heterozygous SOX10 mutation. Patients with SOX10 mutations are additionally at risk for other neurologic abnormalities including seizures, ataxia, and demyelinating neuropathies. HSCR is associated with severe congenital deafness in the absence of pigment abnormalities [62].
HSCR also occurs in syndromes that are not neurocristopathies. Trisomy 21 increases the risk of HSCR by 40-fold (0.8 % of individuals with trisomy 21 have HSCR) and is by far the most frequent chromosomal abnormality identified in HSCR patients, involving 2–10 % of HSCR. HSCR in patients with trisomy 21 shows a more pronounced male predominance and is primarily S-HSCR [63]. Interestingly, the T allele in RET intron 1 enhancer discussed above appears to play a role in the expression of HSCR in trisomy 21 as well as sporadic, non-syndromic HSCR. While the incidence of the T allele is higher in individuals with trisomy 21 HSCR than in individuals with trisomy 21 alone, it is less than that observed in individuals with HSCR alone. This suggests interaction between RET and chromosome 21 genes, perhaps through a reduced HSCR threshold conferred by the extra chromosome 21 [64].
Mowat–Wilson syndrome includes microcephaly, epilepsy, facial dysmorphism, and severe mental retardation. Sixty percent of affected individuals have HSCR. The syndrome is caused by heterozygous de novo inactivating mutations of ZEB2 [65]. Goldberg–Shprintzen syndrome includes microcephaly, polymicrogyria, facial dysmorphism, cleft palate, iris coloboma, and moderate mental retardation. It is caused by mutation in the gene encoding the kif-1 binding protein (known as KBP or KIAA1279) [67]. Animal models suggest that this protein is required for axonal outgrowth in the central and peripheral nervous system and for axonal maintenance [67]. Drevillon et al. demonstrated that KBP protein interacts with microtubules and actin filaments, suggest that the multiple developmental anomalies seen in Goldberg–Shprintzen syndrome might originate from disruption of cytoskeletal homeostasis [68].
Bardet–Biedl syndrome (BBS) includes progressive pigmentary retinopathy, hypogonadism, renal abnormalities, mild mental retardation, obesity, and postaxial polydactyly of the hands and feet. HSCR is reported in several cases. It is caused by at least 14 different genes all of which are involved in the function of primary cilia [69]. As with trisomy 21, PHOX2B and EDNRB mutations, the presence of the T allele of RET associated with expression of the aganglionosis phenotype despite independent biochemical signaling pathways [61]. McKusick–Kaufman syndrome is a rare condition allelic to BBS that includes hydrometrocolpos, postaxial polydactyly, and congenital heart defects. HSCR is reported in 10 % of cases [70].
Smith–Lemli–Opitz syndrome is characterized by growth retardation, microcephaly, severe mental retardation, dysmorphic facies, hypospadias, and syndactyly of the toes. A high percentage of patients also have HSCR. The syndrome is due to mutation in a gene involved in cholesterol metabolism, 7-dehydro-cholesterol reductase [71]. HSCR occurs with limb anomalies in several other rare syndromes. See Table 18.2 for more on the genetics of isolated and syndromic forms of HSCR.
Table 18.2
Genetics of isolated and syndromic forms of Hirschsprung ’s disease
Gene | Mutation | Phenotype |
---|---|---|
A. Isolated Hirschsprung | ||
RET | Heterozygous loss-of-function of tyrosine kinase receptor (many identified) | Long-segment or total-colonic disease more common |
EDNRB | Heterozygous loss-of-function of G protein-coupled receptor | Generally produces short-segment disease |
B. Syndromic Hirschsprung | ||
RET | Heterozygous mutations of cysteines producing constitutive dimerization and activation of the receptor | MEN2A: medullary thyroid carcinoma, pheochromocytoma, parathyroid hyperplasia |
Phox2B | Heterozygous loss-of-function mutation of transcription factor, polyalanine expansion most common | Congenital central hypoventilation syndrome : abnormal autonomic respiratory control, frame-shift mutations increase risk of neuroblastoma |
EDNRB | Homozygous loss-of-function mutation of G protein-coupled receptor | Waardenburg syndrome type 4: pigment abnormalities, deafness. Sox10 mutations also associated with ataxia, neuropathies, and seizures |
ZEB2 | Heterozygous loss-of-function mutation of transcription factor | Mowat–Wilson syndrome: microcephaly, epilepsy, dysmorphic face, cognitive impairment |
KIAA1279 | Homozygous loss-of-function in protein involved in microtubule organization | Goldberg–Shprintzen syndrome: microcephaly, polymicrogyria, dysmorphic face, cleft palate, iris coloboma, mild cognitive impairment |
BBS genes | Homozygous loss-of-function in proteins involved in primary cilia | Bardet–Biedl syndrome: obesity, renal abnormalities, polydactyly, retinitis pigmentosa, hypogonadism, cognitive impairment |
DHCR7 | Homozygous loss-of-function of enzyme in cholesterol production pathway | Smith–Lemli–Opitz syndrome: microcephaly, dysmorphic face, hypotonia, syndactyly, polydactyly, ambiguous genitalia, poor growth |
C. Modifying genes | ||
RET | T allele: single nucleotide substitution in an enhancer sequence | This common allelic variant increases the penetrance of Hirschsprung disease in those with other genetic susceptibilities, like trisomy 21, mutations in EDNRB, Phox2B, and BBS genes |
Chronic Intestinal Pseudo-obstruction
Chronic intestinal pseudo-obstruction (CIPO) is a heterogeneous group of rare primary and secondary disorders in which ganglion cells are present throughout the GI tract in a patient with severe failure of intestinal propulsive motility. The anatomic correlates of CIPO are most often absent, subtle, subjective, or nonspecific. Most cases are sporadic and non-syndromic, but familial and syndromic forms exist. CIPO is generally divided into three groups: neuropathic, mesenchymopathic, and myopathic, depending upon whether predominant abnormalities are found in the enteric nervous system, Interstitial Cells of Cajal (ICC) , or intestinal smooth muscle, respectively. While a genetic basis is suspected in a large percentage of CIPO, it is established in only a small minority of cases.
Neuropathic
Intestinal Neuronal Dysplasia type B (IND B) is characterized by hyperplasia of the submucosal and mucosal portions of the enteric nervous systems, presents with chronic constipation in the first 6 months of life, and is reported in the proximal gut of some individuals with HSCR [72, 73]. Children with isolated IND B often improve in GI function over time with conservative treatment and do not progress to CIPO [74]. While the diagnosis of IND B remains controversial, several animal models and its association with HSCR suggest a genetic cause. IND-like hyperplasia of submucosal ganglia occurs in the proximal gut of EDN3-deficient mice [75] and in the small intestine and colon of apparently healthy EDNRB heterozygous rats [76], both models of HSCR. Attempts to identify mutations in EDNRB in IND B patients have been unsuccessful [77] but Swaminathan et al. recently described IND B-like submucosal ganglion hyperplasia in the proximal margins of HSCR resections [78].
IND A is a rare, fatal syndrome of aplasia or hypoplasia of the enteric sympathetic nerves which presents in the immediate neonatal period with a tonically contracted intestine [79]. The genetics of the disorder are unknown.
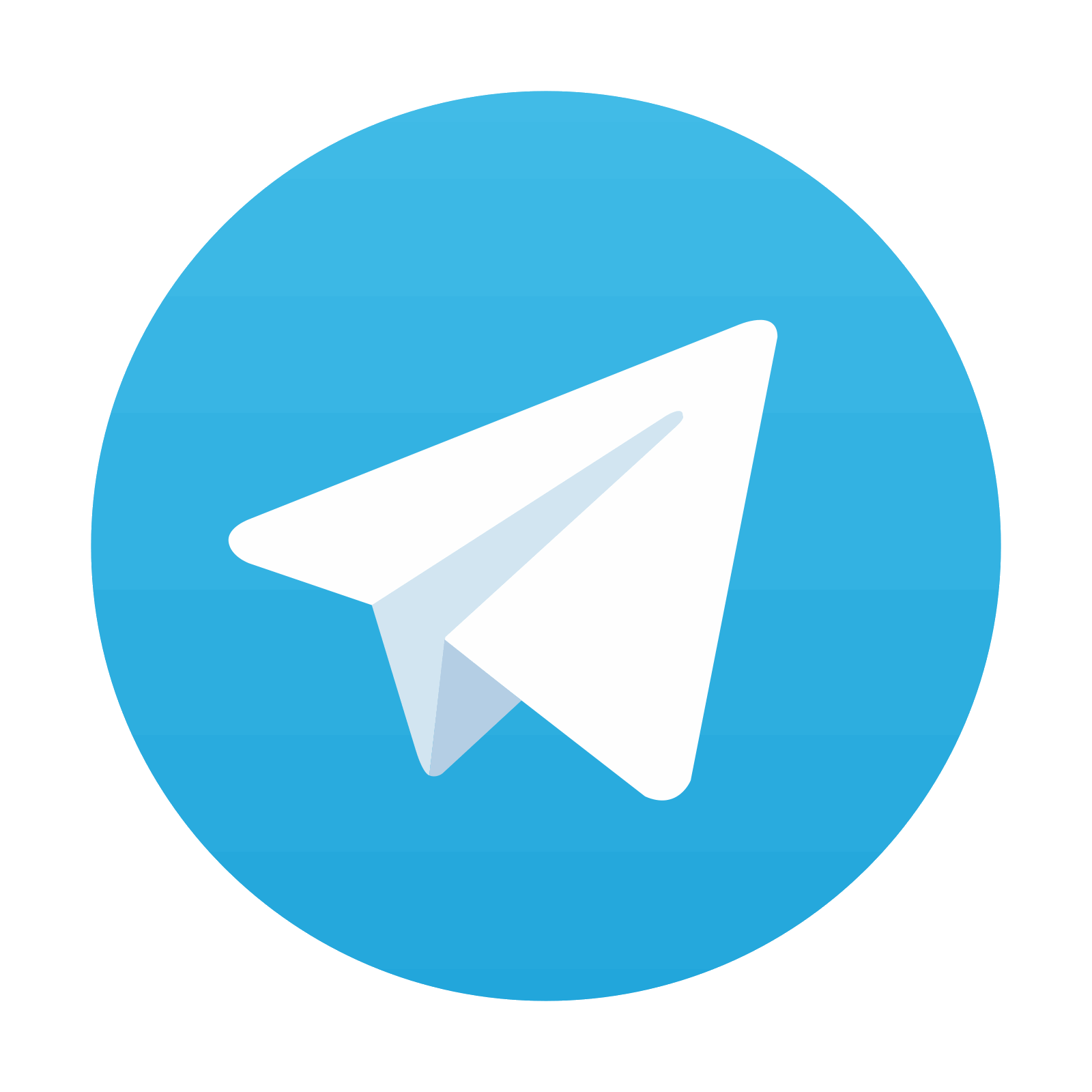
Stay updated, free articles. Join our Telegram channel
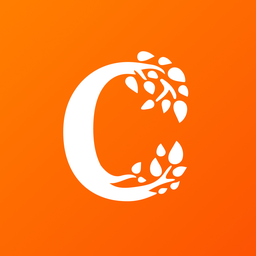
Full access? Get Clinical Tree
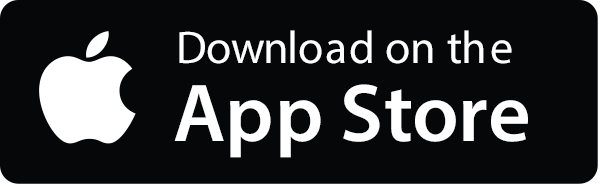
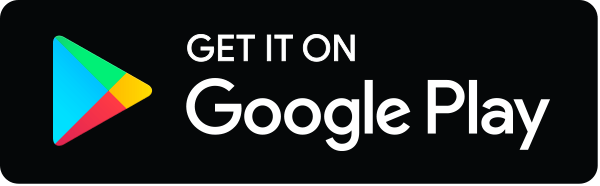