Gastric electrical activity recorded from electrodes on the serosa at various positions from the fundus to the antrum (A–D). Slow waves are generated in the pacemaker region along the greater curvature, then propagate distally and circumferentially (dotted lines with arrowheads). Electrode A, positioned at the fundus, has no slow-wave activity. Electrodes B-D show 3-per-minute depolarizations, indicating slow waves. (Modified from Koch [1].)

Schematic representation of gastric electrical potentials. Intracellular recordings of slow waves show an initial upstroke in potential (1), followed by a plateau potential (2), then a return to baseline. Corresponding extracellular recordings during a slow wave show initial change in potential (3), followed by a return to baseline (4). Note that the slow wave has no corresponding change in tension (i.e., no muscle contraction). In contrast, action potentials are associated with changes in muscle tension. The plateau potential of an action potential is much higher than in the slow wave. Further, the extracellular recording during an action potential shows a downward deflection (5). (From Kim and Malagelada [2])
The frequency and force of contractions associated with slow waves differ based on the region of the stomach in which the smooth muscle is found. This is because smooth muscle cells in different regions exhibit unique electrical characteristics. One key difference is the resting membrane potentials, ranging from −48 to −75 mV. Smooth muscle cells from the corpus to the pylorus have resting membrane potentials that are lower (more negative) than the contraction threshold (about −50 mV). This allows muscle relaxation at baseline with contractions caused by slow waves and/or neurohormonal stimuli.
In contrast, the resting membrane potential of fundic smooth muscle cells is higher (less negative) than the threshold required for contraction. This allows fundic cells to sustain contraction and maintain continuous fundic tone during fasting. It also facilitates a high sensitivity to inhibitory and excitatory stimuli when relaxation or further contraction, respectively, of the fundus is required. When food is swallowed, inhibitory vagal stimulation causes hyperpolarization below −50 mV, thus allowing the fundus to relax and accommodate the increase in intragastric volume.
Interstitial Cells of Cajal
ICCs are the “pacemaker cells” of gastric motility [5–7]. While the exact mechanism of ICC automaticity is still unknown, evidence suggests that Ca2+-activated Cl− channels may be involved [3].

Anatomical location of interstitial cells of Cajal (ICC) in relation to smooth muscle layers and neurons of the enteric nervous system (ENS). Myenteric ICCs (MY-ICCs), located in the myenteric plexus, spontaneously generate slow waves. Slow waves propagate to smooth muscle cells via gap junctions (curved arrow), causing depolarization. Intramuscular ICCs (IM-ICCs) are found within the circular layer of smooth muscle. They are involved in the mediation of neural signals within the ENS (short arrows) and in the transmission of slow waves from MY-ICCs to circular muscles. IM-ICCs also communicate directly with smooth muscle cells via gap junctions. Because of these connections, neural stimulation from the ENS can propagate from the IM-ICCs to smooth muscle cells, then to the MY-ICC network. This allows the ENS to modulate both the timing and amplitude of slow-wave depolarizations; excitatory ENS signals increase chronotopy and amplitude, while inhibitory ENS signals can stabilize membranes and decrease slow-wave depolarization. (Modified from Koch [10])
MY-ICCs are considered the true pacemaker cells because they spontaneously depolarize to create slow waves. Slow waves then propagate through the ICC network via gap junctions. These connections allow slow waves to activate L-type Ca2+ channels in smooth muscle cells, potentially causing contraction [11]. Gap junctions also help maintain the pacemaker frequency (3 cpm) because they allow slow waves generated in the pacemaker region to reach more distal ICCs that have a slower rate of automaticity. When slower ICCs are depolarized by more proximal slow waves, they are prevented from generating their own dyssynchronous slow waves and the pacemaker frequency is maintained.
Smooth muscle cells lack the ion channels necessary for regenerating slow waves. As such, IM-ICCs are required to facilitate the spreading of slow waves from the MY-ICCs to the adjacent layers of circular smooth muscles [12]. From the corpus to the pylorus, IM-ICCs coordinate slow waves and their corresponding muscle contractions. Because the fundus does not have slow waves that need to spread through the muscle layers, fundic IM-ICCs play a different role. Fundic IM-ICCs interact with afferent and efferent fibers of the vagus nerve in order to facilitate receptive relaxation and accommodation. They function as mechanoreceptors with interconnections to vagal afferent neurons and are also innervated by efferent inhibitory vagal fibers that promote smooth muscle relaxation [13].
Nervous System Innervation
While the ICC network displays automaticity independent of external innervation, it is also influenced by neural stimuli. Neural stimuli to the MY-ICCs can modulate the rate of automaticity and the amplitude of depolarizations, thus modifying the pacemaker frequency and the force of peristaltic contractions.
Enteric Nervous System
The enteric nervous system (ENS) consists of neurons in the submucosal and myenteric plexuses in the gut wall. Neurons in this system are both excitatory (acetylcholine, serotonin, substance P) and inhibitory (nitric oxide, vasoactive intestinal polypeptide).

Local reflex circuits of the ENS. Sensory neurons in the mucosa (dashed lines) relay information about the gastric wall to interneurons in the myenteric plexus. Interneurons then stimulate efferent neurons in the submucosal plexus, leading to a change in gastric secretomuscular function. The ENS interacts closely with neurons of the sympathetic and parasympathetic nervous systems (red lines), which can modulate the ENS response to afferent stimuli. Sensory neurons also relay information directly to the CNS to further integrate the secretomuscular response. (From Hall [14])
Parasympathetic Innervation

Distribution of the vagus nerve in the thorax and proximal stomach. The left vagus nerve courses laterally to the proximal esophagus, transitioning anteriorly at the distal esophagus and becoming the anterior vagus nerve. Similarly, the right vagus nerve courses laterally until the distal esophagus, at which point it becomes the posterior vagus nerve (not shown). This transition occurs as the result of a 90° rotation of the stomach during fetal development. (From Drake et al. [19])

Vagovagal reflex neurocircuitry . Vagal afferent fibers (yellow) relay sensory information from the upper GI tract to the tractus solitarius (TS) of the medulla, the cell bodies of which are located in the nucleus tractus solitarius (NTS). NTS neurons integrate the sensory information and then relay it to the adjacent dorsal motor nucleus of the vagus (DMV). Preganglionic parasympathetic neurons of the DMV use ACh to stimulate nicotinic receptors on postganglionic ENS neurons and/or ICCs. Vagal motor activation of postganglionic fibers can induce excitatory effects if the postganglionic fiber releases ACh or inhibitory effects if the postganglionic fiber releases nonadrenergic noncholinergic (NANC) neurotransmitters such as nitric oxide (NO) or vasoactive intestinal polypeptide (VIP). The vagovagal reflex pathway is involved in several GI responses, including receptive relaxation. (Modified from Travagli and Anselmi [21])
Sympathetic Innervation
Sympathetic innervation of the stomach originates from the T6 to T8 spinal nerves. Preganglionic fibers synapse with postganglionic neurons at the celiac ganglion. Postganglionic fibers then travel with the blood supply to innervate the stomach.
The sympathetic nervous system has both direct and indirect effects on gastric motility. Sympathetic stimulation acts directly on the pylorus (as well as other sphincters), causing it to constrict and preventing GI transit into the duodenum. Indirectly, sympathetic stimulation inhibits ACh release from neurons of the ENS to further reduce motility [22].
Gastric Motility in the Fasting State
In the fasting state, slow waves generated by the ICCs cause a cyclic pattern of motility that propagates distally toward the antrum. This pattern is termed the migrating motor complex (MMC) . Each MMC occurs in four phases, all of which recur every 90–120 minutes during fasting. Phase 1 is a period in which virtually none of the slow-wave depolarizations reach the contraction threshold, and little to no contractile activity is present. Phase 2 is characterized by random contractions. In phase 3, regular, high-amplitude contractions occur at a rate of three per minute (the pacemaker frequency). Phase 3 contractions occur in 5- to 10-minute bursts, also known as the “activity front.” Over the course of 90–120 minutes, the activity front migrates from the antrum to the distal ileum. If fasting continues, another activity front will begin at the antrum at the end of the 90- to 120-minute period. Contractions during phase 3 allow gastric emptying of fibrous, nondigestible solids. Phase 4 is characterized by a rapid decrease in contractions prior to the reappearance of phase 1. The MMC pattern stops immediately once food is ingested [10, 23].
Regulation of the MMC pattern is incompletely understood, though it is likely multifactorial. The neurons of the ENS appear to have a significant role in the generation of contractions during phases 2 and 3. During phase 1, slow waves are present, but there is no concurrent excitation from ENS neurons. Excitation begins intermittently in phase 2, then regularly in phase 3, which depolarizes smooth muscle cells to the contraction threshold. These ENS neurons are hypothesized to fire based on an intrinsic timing mechanism that is inhibited by the presence of food in the upper GI tract [24].
Motilin , a polypeptide hormone produced by endocrine cells of the duodenum and jejunum, also plays an important role in the regulation of MMCs . In both humans and dogs, administration of exogenous motilin induces premature phase 3 contractions [25, 26]. In humans, plasma motilin levels fluctuate during fasting and peak during phase 3 of the gastric MMC [27]. The release of motilin is regulated by unclear mechanisms; however, bile acids appear to be involved. Levels of bile acids correlate with fluctuations in plasma motilin levels during the fasting state [28]. Further, postcholecystectomy patients have shown a decreased frequency and shorter duration of phase 3 contractions when compared to healthy volunteers [29]. These findings indicate a possible link between gallbladder emptying and the origin of the MMC [24].
Gastric Motility in the Fed State
The fed state is characterized by prolonged periods of contractile activity in which peristaltic contractions occur more frequently but are less powerful than contractions associated with MMCs. Fed state motility results from the coordination of slow waves, intrinsic and extrinsic innervation, hormonal inputs, and the type/caloric content of the ingested substances.
Gastric Response to Ingestion of Solid Foods
Receptive Relaxation

Summary of gastric motility after ingestion of a solid meal. The fundus initially relaxes in order to accommodate the increase in gastric contents. The fundus then contracts to propel ingested food into the corpus/antrum. Once in the corpus/antrum, food is triturated by recurrent peristaltic waves to produce chyme. Antral peristaltic contractions, indicated by the ring in the above diagram, then empty chyme through the pylorus and into the duodenum. Antropyloroduodenal coordination occurs when the antral contraction occurs in conjunction with pyloric and duodenal relaxation, thus maximizing the efficiency of emptying. (Modified from Koch [30])
Trituration
When food is ingested , excitatory vagal stimulation induces action potentials in the distal stomach, thus replacing the MMC pattern of the fasting state with regular peristaltic waves. Like contractions associated with MMCs, peristaltic contractions occur at a rate of three per minute. However, unlike MMC contractions, peristaltic contractions recur continuously throughout the fed state. As contractions in the fundus begin to push accommodated food into the corpus and antrum, peristaltic waves triturate food into small particles. The particles are then mixed with gastric juices in order to produce chyme.
Gastric Emptying
Peristaltic contractions propagate in the direction of the pylorus; however, some of them stop before reaching the terminal antrum. If the peristaltic wave does not reach the terminal antrum, there is no associated pyloric contraction and a larger aliquot of chyme is delivered to the duodenum. If a peristaltic wave does make it to the terminal antrum, it causes contraction and closure of the pyloric sphincter. When the sphincter is closed, only chyme and food particles <1 mm are able to be emptied into the duodenum. As such, the antropyloric region (antral mill) has the ability to discriminate solid particles according to size. Particles larger than 1 mm are reflected back into the antrum to be broken down into a smaller size.

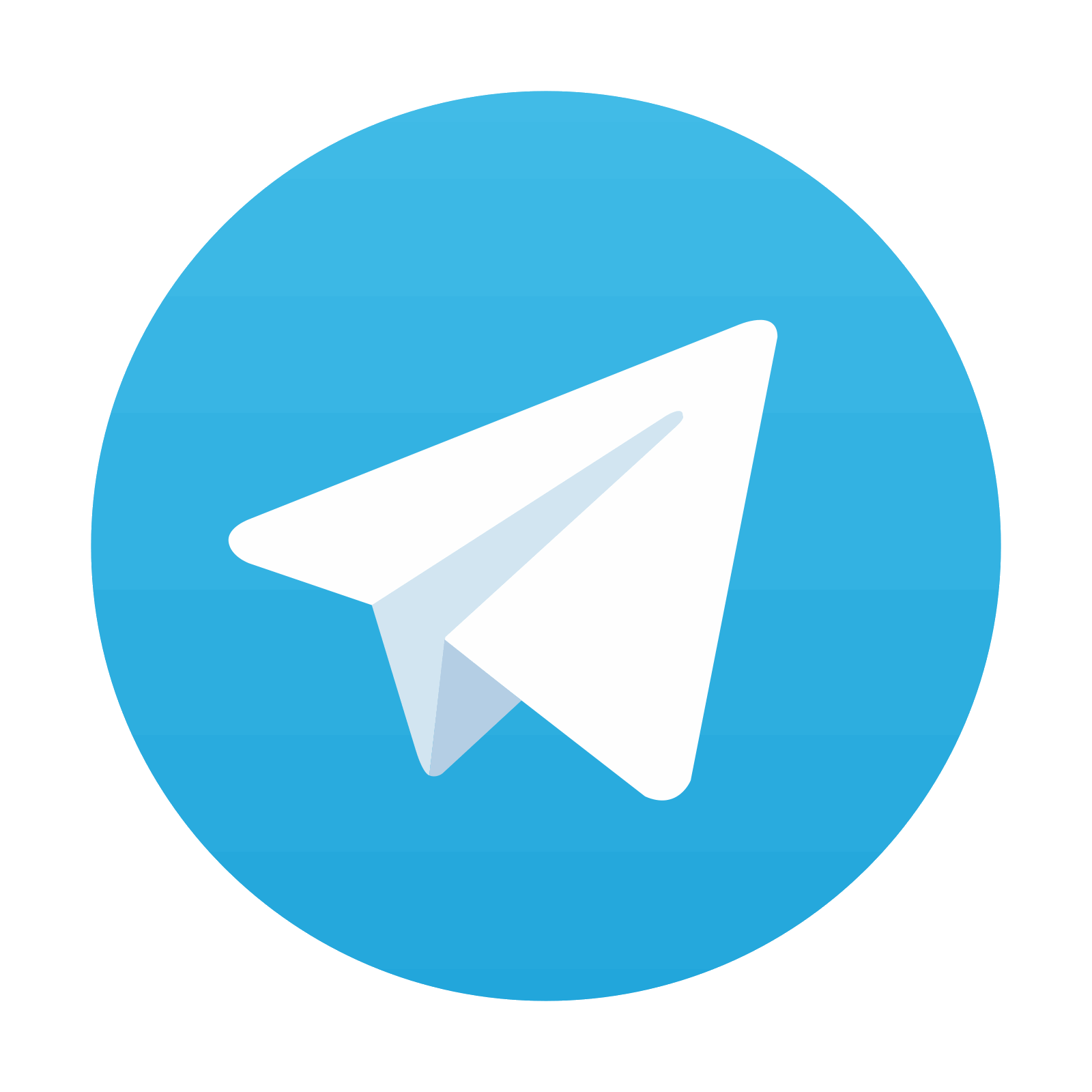
Stay updated, free articles. Join our Telegram channel
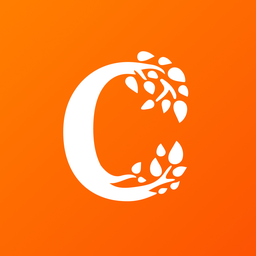
Full access? Get Clinical Tree
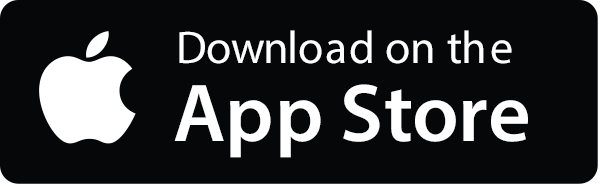
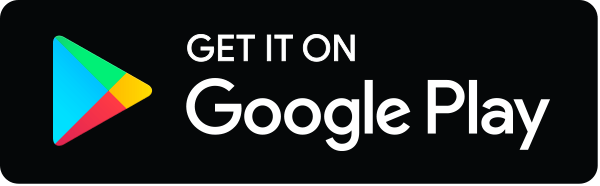