Fig. 12.1
Schematic representation of metabolic changes characterizing dyslipidemia in obesity
It is thought that atherogenic dyslipidemia is caused by a multiple array of metabolic abnormalities such as: (1) increased production of TG-rich lipoproteins from the liver and intestine, (2) increased cholesterol synthesis, (3) delayed clearance of TG-rich lipoproteins, and (4) increased HDL catabolism. However, an important question is which of these abnormalities is the more relevant in determining the typical lipid phenotype of AD. Several lines of evidence indicate that the pivotal role is played by the increased production of very-low-density lipoproteins (VLDL) by the liver. In vivo turnover studies have, in fact, shown that the dominant feature of AD is the increased production rate of VLDL-apo B by the liver, mainly as large VLDL particles (VLDL1) [8]. The VLDL1 production rate is the strongest determinant of TG concentration in the plasma and is significantly related to indices of insulin sensitivity [8].
The enhanced influx of VLDL particles into the bloodstream not only determines hypertriglyceridemia but is the major cause of the other lipid abnormalities of AD since it will lead to delayed clearance of the TG-rich lipoproteins and formation of sdLDL [9, 10]. In the presence of hypertriglyceridemia, the cholesterol-ester content of LDL decreases, whereas that of TG increases due to the enhanced activity of the cholesteryl ester transfer protein (CETP), the circulating enzyme that promotes the exchange of cholesterol esters (CE) with TGs between VLDL and LDL. However, the increased TG content within the LDL is hydrolyzed by hepatic lipase, which leads to the formation of sdLDL particles [11]. The development of sdLDL in obesity is mainly due to increased TG concentrations and does not depend on total body fat mass [12].
Lipolysis of TG-rich lipoproteins is also impaired in obesity by reduced activity of lipoprotein lipase (LPL), the enzyme that regulated the lipolysis of TGs in VLDL and intestinal-derived chylomicron [13]. Studies using stable isotopes have shown a decreased catabolism of chylomicron remnants in obese subjects with the waist/hip ratio as the best predictor for the fractional catabolic rate [14]. Additionally, prolonged postprandial lipemia leads to elevated levels of FFA, resulting in detachment of LPL from its endothelial surface [15], further reducing the postprandial catabolism of TG-rich lipoproteins. It has been well demonstrated that postprandial hyperlipidemia with accumulation of atherogenic remnants is especially linked to visceral obesity [16]. Van Oostrom et al. have shown that diurnal triglyceridemia in obese subjects correlates better to waist circumference than to body mass index [17], which is in agreement with the hypothesis that the distribution of adipose tissue modulates postprandial lipemia. There are reports suggesting that also the metabolism of LDL might be altered in obese individuals mainly due to the reduced expression of LDL receptors [18].
The increased number of remnants of chylomicrons and VLDL together with impaired lipolysis in obesity also significantly affects the HDL metabolism. As mentioned before, the increased number of TG-rich lipoproteins results in increased CETP activity, which exchanges CE from HDL for TG from VLDL and LDL [19]. Moreover, lipolysis of these TG-rich HDLs occurs by hepatic lipase resulting in small HDL with a reduced affinity for apo A-I, which leads to dissociation of apo A-I from HDL. This will ultimately lead to lower levels of HDL-C and a reduction in circulating HDL particles with impairment of reversed cholesterol transport [20].
12.3 The Atherogenic Potential of Lipoprotein Abnormalities in Obesity
The resulting effect of all abovementioned abnormalities in the lipoprotein metabolism in obesity is the accumulation of pro-atherogenic lipoproteins and the impairment of HDL function. In fact, sdLDLs are relatively slowly metabolized with a 5-day (instead of 2-day) residence time, which enhances its atherogenicity [11]. In addition, sdLDLs have an increased affinity for arterial proteoglycans resulting in enhanced subendothelial lipoprotein retention [21]. Finally, it has been described that sdLDLs are more susceptible to oxidation, in part due to less free cholesterol and anti-oxidative content [19]. Remnants of chylomicrons and VLDL are also involved in the development of atherosclerosis. Several investigations have documented an association between TG-rich lipoproteins and remnant cholesterol levels with the presence of coronary atherosclerosis [22]. This has been explained by the fact that chylomicron remnants and LDL may migrate into the vessel wall and become trapped in the subendothelial space where they can be taken up by monocytes/macrophages [23]. This mechanism may be facilitated by the fact that subendothelial remnants of chylomicrons and VLDL do not need to become modified to allow uptake by scavenger receptors of macrophages in contrast to native LDL [23]. Even though LDL particles migrate more easily than chylomicron remnants into the subendothelial space, the number of migrated particles does not necessarily translate into more cholesterol deposition since chylomicron remnants contain approximately 40 times more cholesterol per particle than LDL [23]. Other mechanisms of remnant-mediated atherogenesis, which may play a role in obesity, comprise the postprandial activation of leukocytes, generation of oxidative stress, and production of cytokines [24]. On the other side, the abnormality in HDL concentration and composition is thought to have an important impact in deteriorating the contribution of this particle to the cholesterol efflux from the cells. The capacity of cholesterol efflux, which is the first step in the reverse cholesterol transport, has been associated with increased risk of coronary artery disease and with the flow-mediated vasodilation in diabetic obese patients [25]. It has been also reported that HDL isolated from obese diabetic patients shows a reduced capacity to promote ex vivo cholesterol efflux from cells and that this may be related to a lower expression of ABCA1, which is the membrane transporter responsible for the first step of transfer of cholesterol from cell membrane to HDL particle [26].
12.4 Lipid Targets for Treatment of Dyslipidemia in Obesity
The EAS/ESC guidelines recommend testing lipids in obese subjects in order to assess their cardiovascular risk [27]. However, the necessity to initiate pharmacological treatment next to lifestyle intervention in obese subjects with dyslipidemia depends on the comorbidity, the potential underlying primary lipid disorders, and the calculated cardiovascular risk [13, 27]. Irrespective of increased body weight, LDL-C is the primary target for the treatment of dyslipidemia in obesity [27] (Table 12.1). Nevertheless, the presence of obesity can affect treatment targets since obesity may contribute to increased remnant cholesterol, higher TG levels, and lower HDL-C concentrations. Therefore, apo B or non-HDL-C levels are recommended as secondary treatment targets next to LDL-C levels in the presence of AD [13, 27]. Apo B represents the total number of atherogenic particles (chylomicrons, chylomicron remnants, VLDL, IDL, and LDL), whereas non-HDL-C represents the amount of cholesterol in both the TG-rich lipoproteins and LDL. Recently, a meta-analysis has shown that implementation of non-HDL-C or apo B as treatment target over LDL-C would prevent an additional 300,000–500,000 cardiovascular events in the US population over a 10-year period [28]. However, others did not describe any benefit of apo B or non-HDL-C over LDL-C levels to assess cardiovascular risk [29]. The treatment target for non-HDL-C should be 30 mg/dl higher than the target for LDL-C, which corresponds with non-HDL-C levels of 160 mg/dl and 130 mg/dL for subjects at moderate and high risk, respectively. Treatment targets for apo B are approximately 0.80–1.00 g/L [27]. Specific treatment targets for TG levels are unavailable, especially since TGs are highly variable and increase during the day. However, pharmacological interventions to lower specifically TG should be initiated when TG levels exceed 800 mg/dl to reduce the risk for pancreatitis [13, 30].
Table 12.1
Targets, therapeutic goals, and suggested strategies to control dyslipidemia in patients with obesity
Targets | Parameters | Therapeutic goals | Suggested strategies |
---|---|---|---|
Primary target ↓ LDL | LDL-C | High risk <100 mg/dl | Statin as first choice |
Moderate risk <130 mg/dl | Titrate according to guidelines | ||
Lower risk <160 mg/dl | |||
Secondary target ↓ non-HDL | Non-HDL-C | High risk <130 mg/dl | Intensify statin therapy |
Moderate risk <160 mg/dl | Consider adding fibrate mainly in high-risk individuals. Consider PUFA | ||
Lower risk <190 mg/dl | If TG <500 mg/dl initiate with fibrates before adding statins | ||
Tertiary target ↑ HDL | HDL-C | Therapeutic goals “>40 mg/dl” | Maximize dietary intervention |
Increase physical activity | |||
Consider adding fibrates after LDL-lowering drugs |
12.5 Pharmacological and Non-pharmacological Interventions for Dyslipidemia in Obesity
Treatment of obesity-associated dyslipidemia should be focused on lifestyle changes including weight loss, physical exercise, and a healthy diet. Lifestyle changes synergistically improve insulin resistance and dyslipidemia [31]. Weight loss has been demonstrated to markedly reduce fasting and non-fasting TG concentrations, which can be attributed to an increase in LPL activity, and thereby an increased catabolism of TG-rich lipoproteins [32]. Besides reductions in fasting and non-fasting TG, a small reduction in LDL-C can be expected upon weight loss, which may be attributed to increased LDL receptor activity. A weight loss of 4–10 kg in obese subjects resulted in a 12 % reduction in LDL-C and a 27 % increase in LDL receptor mRNA levels [33]. The type of dietary fat also affects postprandial lipemia. In obese men, a moderate weight loss (approximately 10 %) induced by a diet low on carbohydrates and saturated (SFA) and high on monounsaturated fatty acids (MUFA), resulted in a 27–46 % reduction in postprandial TG levels [34]. Long-term intervention with MUFA resulted in a reduction in postprandial inflammation when compared to a diet rich in SF in patients with metabolic syndrome (MetS) [35].
Physical exercise has been shown to increase LPL and hepatic lipase activity, which stimulates TG lipolysis [36]. The mechanism of exercise-induced LPL activity remains unclear, but it was hypothesized that exercise stimulates especially muscular LPL activity. A 12-week walking program supplemented with fish oil (1,000 mg eicosapentaenoic acid and 700 mg docosahexaenoic acid daily) in subjects with the MetS resulted in lower fasting TG and decreased the postprandial response of TG and apoB48 [37]. More interestingly, physical activity has been reported to favorably influence ectopic fat accumulation (mainly in the liver) in obese individuals. Exercise training for 16 weeks in obese subjects with non-alcoholic fatty liver disease (NAFLD) resulted in a small reduction in intrahepatic TG content, although no changes in VLDL-TG or apoB100 secretion were observed [38]. Exercise-induced reductions in intrahepatic TG content have also been reported even in the absence of weight loss [39]. Moreover, intrahepatic TG content was reduced in overweight men after a low-fat diet for 3 weeks, whereas a high-fat diet increased intrahepatic TG [40]. The plasma TG-lowering effect of exercise and weight loss is the most consistent finding in studies concerning blood lipids [41], whereas increasing HDL-C levels by exercise remains controversial, especially in those subjects with high TG and low HDL-C levels [42]. Other dietary factors such as dietary fibers have been shown to improve nutrient absorption and have also been linked to insulin metabolism. Daily intake of resistant starch from bread, cereals, vegetables, and pastas is approximately 5 g/day in the Western world, which is highly insufficient for potential health benefits [43]. Recently, a randomized study in 15 insulin-resistant subjects has shown that 8 weeks of resistant starch supplementation (40 g/day) improved insulin resistance and subsequently FFA metabolism. Resistant starch ingestion resulted in lower fasting FFA concentrations and increased TG lipolysis by enhanced expression of genes like LPL coupled with increased FFA uptake by skeletal muscle [44]. However, no effect of resistant starch supplementation was observed on TG and cholesterol concentrations [44].
Unfortunately, lifestyle modifications are often insufficient to achieve weight loss and improvement of dyslipidemia, and, therefore, pharmacological treatment must be considered. The effects on dyslipidemia of antiobesity drugs are very limited, if present. A recent meta-analysis concerning antiobesity drugs reported a mean weight loss of 3.13 kg, but a very little improvement of dyslipidemia [45]. Orlistat, which reduces the lipolysis of TG within the gastrointestinal system and thus prevents absorption of intestinal fat by 30 %, showed only a modest reduction in LDL-C of 8 mg/dl. Sibutramine, which increases the sensation of satiety by modulating the central nervous system, showed a 12 mg/dl reduction in TG, whereas rimonabant did not show any lipid improvements [45]. Conversely, bariatric surgery-induced weight loss has been associated with decreased TG and increased HDL-C levels [46].
Obesity-associated dyslipidemia may well be treated with specific hypolidemic medications (Table 12.1). Statins are the first-choice agents to reduce LDL-C, non-HDL-C, and/or apo B. However, statins lower TG only marginally and do not fully correct the characteristic dyslipidemia seen in obesity, which may contribute to the residual risk after initiating statin therapy [47]. Statins inhibit the enzyme 3-hydroxy-3-methylglutaryl-coenzyme A (HMG-CoA), which is the rate-limiting step in the hepatic cholesterol synthesis. This, in turn, increases the fractional catabolic rate of VLDL and LDL together with a slight reduction in hepatic secretion of VLDL. Therefore, statins lower both remnant cholesterol and LDL-C levels [48]. Recently, strategies for combination therapies with statins to achieve even lower cholesterol levels have been reviewed [47]. Combinations can be made with ezetimibe, which inhibits the intestinal cholesterol absorption by interaction with NPC1L1, which results in an additional 20 % lowering effect on LDL-C, but without affecting TG or HDL-C concentrations [49]. On the contrary, fibrates are primarily indicated in the case of hypertriglyceridemia, and they reduce TG by approximately 30 % and LDL-C by 8 %, whereas HDL-C is increased by an average of 9 % [50]. Fibrates are peroxisome proliferator-activated receptor-α agonists, which transcriptionally regulate lipid metabolism-related genes. Fibrates as monotherapy have been shown to reduce cardiovascular mortality, especially in subjects with characteristics of the MetS with TG levels >190 mg/dl [51]. However, there is controversy about the effectiveness of fibrate therapy on top of statin therapy since the ACCORD trial was unable to confirm a beneficial effect on cardiovascular end points by fenofibrate combined with statins in diabetic patients [52]. Nevertheless, It must be mentioned that subgroup analyses suggested a beneficial effect of combination therapy of fibrates with statins in patients with AD [52]. Omega-3 fatty acids, which decrease the hepatic synthesis and accumulation of TG [53], have been shown to reduce plasma TG by 25–30 % by effectively reducing the hepatic secretion of VLDL in insulin-resistant subjects [54]. Omega-3 fatty acids have also been shown to increase the conversion of VLDL into IDL, which suggests an additional benefit for combining omega-3 fatty acids with statins by increased catabolism of VLDL, IDL, and LDL. Drugs that increase insulin sensitivity like metformin or thiazolidinedione derivatives have no or minimal effects on lipoprotein profile in obesity [55].
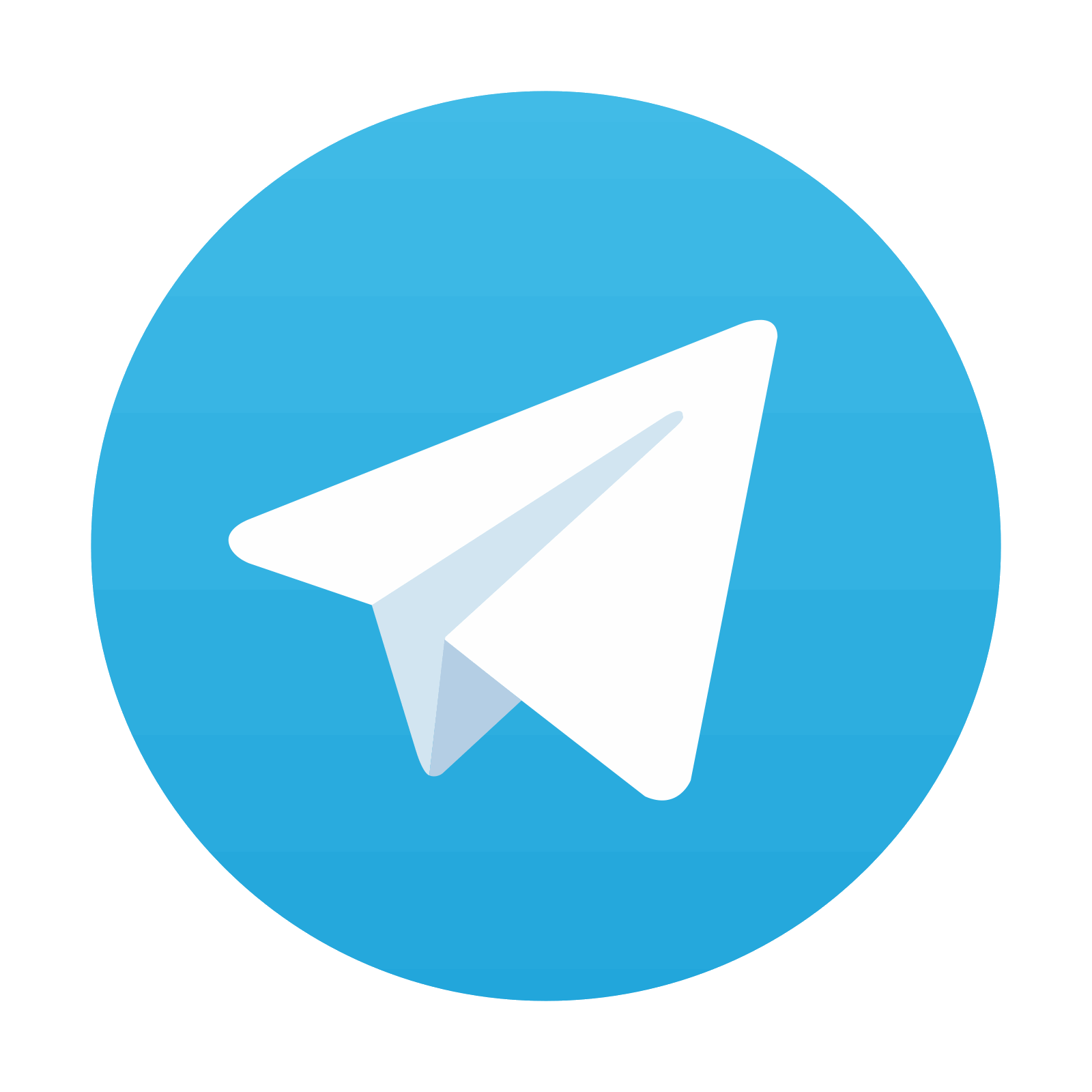
Stay updated, free articles. Join our Telegram channel
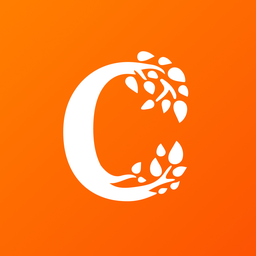
Full access? Get Clinical Tree
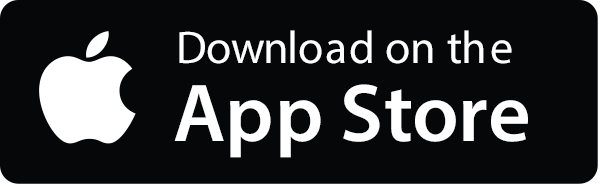
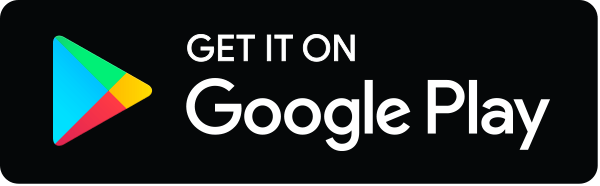