Fig. 2.1
Development of enteric nervous system, smooth muscle and interstitial cells of Cajal within the developing human gut. (a) At week 11 of development, αSMA-staining (green) is apparent in the circular (cm) and longitudinal (Lm) muscle layers, located on either side of the p75NTR-positive (red) cells (the neural crest-derived cells, arrows) of the presumptive myenteric plexus. Occasional areas of p75NTR immunoreactivity are also apparent in the region internal to the circular muscle layer, corresponding to the presumptive submucosal plexus (double arrowheads). (b) At week 12, the circular (cm) and longitudinal (Lm) muscle layers are strongly immunopositive for αSMA. Between the muscle layers, p75NTR labelling is present in groups of cells comprising myenteric ganglia (arrows). (c) At week 14, αSMA labelling is strong in the circular (cm) and longitudinal (arrowhead, Lm) muscle layers and weak in the muscularis mucosae (mm), adjacent to the villi (v). The walls of blood vessels within the submucosa are also immunopositive for αSMA (asterisks). p75NTR staining is present within ganglia of the myenteric plexus (arrows) and in nerve fibres within the submucosa (double arrowheads). (d) At week 11, Kit immunostaining is widespread within the developing smooth muscle layers, particularly surrounding (arrows) the presumptive myenteric ganglia (asterisks). At week 12 (e) and week 14 (f), Kit-positive ICC (arrows) is restricted to the areas surrounding ganglia (asterisks). Scale bar = 50 μm (a, b); 100 μm (c). (From Wallace, A.S. and Burns, A.J. (2005) Development of the enteric nervous system, smooth muscle and interstitial cells of Cajal in the human gastrointestinal tract. Cell and Tissue Research: 319, 367–382 (modified from Figures 7 and 8, with kind permission of Springer Science + Business Media))
Peristaltic function of the gut requires the development of the contractile apparatus of the smooth muscle cells, which enables the cell to tense and relax, thus generating the contractile motion. The contractile apparatus is comprised of bundles of actin and myosin filaments (myofilaments), attached to the cell membrane via actin-rich dense bodies (the functional equivalent of Z lines in skeletal muscle). Upon receipt of contractile stimulus and signal activation, the myosin (thick) filaments slide over the actin (thin) filaments to produce cellular contractions [8]. Myofilaments are oriented in parallel arrays along the long axis of the smooth muscle cells and cause shortening along this axis. Studies of chick embryonic intestine demonstrate that upon aggregation and elongation of muscle precursors, the first indications of the developing contractile apparatus are evident as thin bundles of actin filaments, which are initially unattached to the cell membrane [5]. Several days later, thick myosin filaments form, which are also unattached to the cell membrane. Soon after birth, however, extensive insertion of the contractile apparatus to the cell membrane is evident, with abundant microtubules oriented parallel to the cell length [5].
Although smooth muscle can undergo spontaneous contractions, coordination of these contractions is regulated through intrinsic innervation by nerves of the ENS (see below). Because smooth muscle cells of the gut are uninuclear, in contrast to multinuclear skeletal muscle cells, neighbouring smooth muscle cells communicate via gap junctions to enable passage of electrical impulses between cells and to allow generation of the coordinated progressive wave contractions characteristic of the gut wall. These gap junctions are observed perinatally in intestinal smooth muscle [5] consistent with the neuronally mediated organised peristaltic activity that commences just before feeding begins at birth [9]. However, ENS- and ICC-independent organised spontaneous contractions can also be observed in mouse intestine several days before birth [10]. Because gap junctions are not observed at these earlier time points, the mechanisms enabling such organised smooth muscle contractions are currently unknown.
Smooth Muscle Development Defects in Motility Disorders
Hirschsprung’s disease (HSCR) is a common developmental disorder characterised by the absence of enteric neurons and glial cells in a variable portion of the distal gut [11–14]. In the aganglionic region of the affected gut, the smooth muscle is tonically or spastically contracted, which leads to bowel obstruction. Why an absence of ENS neurons would lead to such muscle contraction is currently unclear. One hypothesis is that this results from the absence of innervation by fibres from relaxant neurons [15, 16], while an alternative model is that over-proliferation of extrinsic, stimulatory nerve fibres leads to increased contractility of affected segments [17]. Further experimentation will be necessary to distinguish between these and other models and to shed light on why smooth muscle remains contracted in affected segments in HSCR.
Interestingly, in certain mouse models of HSCR , aganglionic segments exhibit an increased thickness of the circular and longitudinal muscle layers [18], and these thicker muscle regions display an increased contractile force [19, 20]. However, defects in muscle layers are not observed in all models of aganglionosis [21, 22]. Thus, muscle hypertrophy may not provide a generally applicable explanation for tonic contraction of affected segments in HSCR or other motility disorders, although it may provide explanation for disease features in some cases.
Defects in smooth muscle characterise some rare cases of chronic intestinal pseudo-obstruction (CIPO) , and these are classified as myopathic CIPO and, like most cases of CIPO, are largely idiopathic [23]. Mouse mutations affecting the development of gut smooth muscle have been identified and include defects in the proliferation of smooth muscle progenitors and radial patterning of the gut [24]. Further studies in mouse or other model organisms are helping to uncover the basic cellular processes required for normal development of smooth muscle and therefore shed light on the genesis of human gut diseases.
The Enteric Nervous System
The smooth muscle of the gut is innervated by intrinsic neurons of the enteric nervous system (ENS). In addition, extrinsic nerves , comprising vagal and spinal afferent neurons that have their cell bodies outside the gut and communicate to the CNS, make axonal connections to ENS neurons and modify their activity [25–28]. Here we focus on the gut intrinsic ENS, which can function independently of the CNS to maintain local reflex activity to control muscular mixing and peristaltic movements, changes in blood flow and secretion of water and electrolytes [25].
The ENS consists of interconnected ganglia, containing neurons and glial cells. Ganglia are organised in two plexus layers which span the length of the gut, an outer myenteric plexus, situated between the longitudinal and circular muscle layers, and an inner submucosal plexus lying between the circular muscle and the muscularis mucosae [25]. ENS neurons function to innervate appropriate target tissues, such as the muscle, the mucosa and the blood vessels of the gut and to create interconnections to other ENS neurons and ganglia as well as to extrinsic neurons. Such a wide spectrum of functional requirements is satisfied by vast numbers (millions of neurons in the small intestine [29]) of different neuronal types [30]. Overall the ENS is estimated to contain more neurons than found in the spinal cord [31]. ENS glial cells are even more numerous (fourfold) than neurons and function as support cells for ENS neurons and may also play a role in modulating neuronal activity or in interactions with other gut cell types such as endothelial cells and intestinal epithelial cells [32]. Following injury, glial cells also can give rise to new neurons [33]. Recently, the diversity of enteric glial cells (EGCs) has been described and includes four morphologically distinct populations that occupy unique niches within the gut and that have distinguishable physiological properties [34]. Moreover, one of these subpopulations, EGCs in the lamina propria of the mucosa, has been shown to be responsive to microbiota in the gut lumen and is replenished as necessary by glial cells that migrate from the underlying plexi [35].
Migration of ENS Precursors
The ENS derives from neural crest cells (NCC) that delaminate from the neural tube and migrate towards the developing gut tube. The primary contribution to the ENS comes from vagal NCC, which begin migration at E9.0–9.5 in the mouse, and by 4 weeks gestation in human, and enter the foregut and move in a rostral-to-caudal direction to colonise the entire gut tube by E15 in mouse and by 7 weeks gestation in human [7, 36, 37] (Fig. 2.1). In addition, trunk neural crest from the posterior vagal region makes a small contribution to the foregut ENS [37], whereas the hindgut receives contribution from the sacral neural crest, which begins their migration at a later stage, exhibits distinct migratory properties and enters and colonises exclusively the hindgut [38–41]. The myenteric ganglia emerge first during development, whereas the submucosal plexus originates later when cells from the myenteric plexus migrate through the circular muscle towards the submucosa [42] and are clearly seen in the submucosal region of the human intestine at 11 weeks of gestation [7] (Fig. 2.1).
A variety of studies have examined the behaviour and pattern of migratory enteric neural crest-derived cells (ENCCs) as they move into and along the developing gut. Time-lapse movies of fluorescently labelled ENCCs, which are rendered fluorescent through dye-labelling of cells or use of transgenic mice possessing fluorescent ENCCs, have enabled their migratory behaviours to be monitored. ENCCs are found to advance through the gut steadily as multicellular strands, with a few isolated cells preceding the migratory wavefront [43–45]. The pattern of advance changes as ENCCs reach the caecum, when the advancing strand pauses and cells separate and adopt a solitary meandering behaviour [44]. After several hours, the cells leave the caecum and continue movement through the hindgut as a network of interconnected cells to complete gut colonisation [43]. More recently, the importance of ENCCs that migrate from the midgut to the hindgut across the mesentery has been uncovered, and such ‘trans-mesenteric’ ENCCs have been shown to form a substantial portion of the hindgut ENS in mouse [46]. Finally, using new technologies to label individual ENCCs in living tissue, the trajectories of single cells have been described, revealing that a balance of non-directional and directional movements of individual cells modulates caudal advance of the population and helps to supply a uniform density of ENCCs to the gut [47]. Interestingly, immature neurons that are being generated even as ENCCs are migrating through the gut (see below) also exhibit rostral-to-caudal migration, albeit more slowly than their ENCC precursors [48]. Approximately half of the immature neurons migrate by caudal movement of cell bodies along long leading processes [48].
Among the signals involved in directing migration of NCC along the gut, perhaps the best understood are the components of the RET pathway [49]. Loss of RET signalling results in gut aganglionosis in mice [50], and the RET gene is the main gene implicated in HSCR in humans [11, 51, 52]. Within the gut, the RET receptor and the glycosylphosphatidylinositol (GPI)-linked GDNF family receptor α1 (GFRα1) co-receptor are expressed on NCC, and the ligand, glial cell line-derived neurotrophic factor (GDNF), is expressed within the gut mesenchyme (Fig. 2.2) and has been shown, in vitro, to be a chemoattractant for NCC [53]. Consistent with this in vitro finding, GDNF is expressed in the stomach when the ENCC wavefront is in the oesophagus and is elevated in the caecum as ENCCs migrate towards this distal part of the gut. Thus it appears that NCC move towards centres of GDNF expression that are upregulated progressively further along the gut. In addition, GDNF expression is observed in a gradient across the mesentery and is proposed to guide the trans-mesenteric ENCC migration of cells colonising the hindgut [46]. More extensive information concerning the molecular mechanisms involved in ENS development can be found in the following reviews [12, 54–58].


Fig. 2.2
Sources, migratory routes and gene expression in neural crest cells contributing to the ENS. (a) At approximately embryonic day (E) 8.5–9 in the mouse, vagal neural crest cells (red arrow) invade the anterior foregut and migrate in a rostral-to-caudal direction to colonise the entire foregut (FG), midgut (MG), caecum and hindgut (HG) and give rise to the majority of the enteric nervous system (ENS, red dots). Colonisation is complete by E15.5. The most caudal vagal neural crest cells, emanating from a region overlapping with the most anterior trunk neural crest cells (blue arrow), make a small contribution to the ENS of the oesophagus and the anterior stomach (blue dots). Finally, sacral neural crest cells (yellow arrow) also make a small contribution, beginning their migration at approximately E13.5 and migrating in a caudal to rostral direction to colonise the colon (yellow dots). (b) As vagal neural crest cells (red) emigrate from the neural tube, they express SRY-box 10 (SOX10) and endothelin receptor-B (EDNRB). (c) Upon entering the foregut at E9–9.5, enteric neural crest-derived cells (ENCCs) begin to express RET. Within the gut mesenchyme, the RET ligand glial cell-line-derived neurotrophic factor (GDNF) is expressed at high levels in the stomach (green) and the EDNRB ligand endothelin 3 (EDN3) is expressed in the midgut and hindgut (pink). (d) As ENCCs migrate caudally at approximately E11, they encounter high levels of GDNF and EDN3 expression in the caecum (yellow). Cells behind the wavefront begin progressive differentiation towards neural and glial cell fates. Beginning at E11.5, GDNF and EDN3 are expressed in the distal hindgut (not shown). (From Heanue and Pachnis (2007) Enteric nervous system development and Hirschsprung’s disease: advances in genetic and stem cell studies. Nat Rev Neurosci 8(6): 466–479. Panel (a) modified, with permission from The Company of Biologists Ltd., from Durbec et al. (1996) Development 122(1): 349–358)
Proliferation in the ENS
The colonisation of the entire gut takes place over many days (E9.0 to E15 in the mouse) and from week 4 to 7 in human gestation [7], and during this period, the gut is growing considerably in length and continues to grow during further embryonic and postnatal stages. In order to continue expansion into caudal gut regions as well as to keep pace with the expanding length of the already colonised gut regions, the relatively small number of ENCCs must therefore increase greatly in number throughout the gut. For that reason, it is not surprising that proliferation of ENCCs is observed and is essentially equivalent at all rostral-caudal positions [59]. Nevertheless, the size of the starting pool of ENS progenitors has a significant impact on the capacity of ENCCs to completely colonise the gut. In a number of experimental conditions in which the initial pool of ENCCs is reduced, there is a failure of ENCC to colonise the distal gut [21, 39, 60] or to appropriately populate the entire gut with ENS neurons [61]. Moreover, mathematical models which suggest that ENCC proliferation is a key driver of colonisation have been substantiated by experimental data [62–64].
Regarding molecular mechanisms influencing ENCC proliferation, the RET ligand, GDNF, has been shown to increase the proliferation rate and numbers of enteric neural precursors in vitro and in vivo [61, 65, 66]. An additional level of control of GDNF/RET signalling is mediated by factors such as those within the endothelin receptor-B (EDNRB) pathway. Activation of EDNRB specifically enhances the effect of RET signalling on the proliferation of uncommitted ENS progenitors [67], and the EDNRB ligand, endothelin-3 (ET-3), which directly regulates ENCC proliferation and differentiation [68], modulates the action of GDNF by inhibiting neuronal differentiation [66]. Another mediator of GDNF/RET signalling is Prokineticin-1 (Prok-1) which has been shown to maintain proliferation and differentiation of ENCCs [69]. Another factor shown to be involved in ENCC proliferation is retinoic acid (RA), which enhances proliferation of subsets of ENS precursors and increases neuronal differentiation [70], and retinaldehyde dehydrogenases, that produce RA, have been shown to be involved in ENS development and function [71].
Differentiation in the ENS
The mature ENS contains a large variety of neuronal cell types and glial cells, with neuronal types distinguishable on the basis of their morphologies, immunohistochemical profiles and electrophysiological properties [30, 72–74]. Even as ENCCs are migrating through rostral gut regions, some of these ENCCs are undergoing neuronal differentiation [45, 75–77], thus beginning the process of generating the wide range of neuronal cell types present in the mature ENS. Nevertheless, ENS progenitor cells persist among the pool of ENCCs, and differentiation of distinct neuronal types continues throughout embryonic and postnatal development [74, 78]. Differentiation of glial cells begins in the late embryonic period, around E15, and continues during the postnatal period [74, 79]. Interestingly, cells expressing early neural differentiation markers can continue to proliferate [59, 75], thus providing an additional mechanism to expand ENS cell number to populate the continuously growing gut.
In order to generate the distinct classes of ENS neurons and glial cells, there is a progression during ENCC development from bipotential ENS progenitor cells, capable of giving rise to both neurons and glial cells, to separate neural and glial progenitor cells (Fig. 2.2) and the further subdivision of neural progenitors into precursors of the distinct neuronal types. While the advancement of cells through the stages of this progressive lineage restriction can be identified using molecular markers [74] (Fig. 2.2), the factors influencing the changes in cell state are largely unknown. Indeed, only a few instances have transcription factors, such as Mash1, which generates some serotonergic neurons [36], or Hand2, which is involved in the development of vasoactive intestinal polypeptide (VIP) neurons [80] and in terminal differentiation [81] been identified. In most cases, genes affecting development of the ENS affect all lineages due to defects in the survival or proliferation of early progenitor cells (reviewed in [54]). The capacity for ENS progenitor cells to be propagated in culture has the potential in the future to complement gene deletion studies in elucidation of the factors controlling progressive ENS lineage restriction.
It has been postulated that defects in the development of specific subtypes of enteric neurons may underline certain motility disorders [30]. Although some ENS neurons of the human myenteric plexus have been characterised [82–84], the cataloguing of ENS subtypes may still be too preliminary to enable motility disorders to be analysed on this basis.
Ganglia Formation and Connectivity in the ENS
Ganglia are the functional units of the ENS. To perform their tasks, they must contain the appropriate number of neuronal subtypes and innervate appropriate targets, i.e. the muscle layers, the mucosa and the blood vessels [25]. Unfortunately, the mechanisms controlling the formation of ganglia, the generation of neuronal diversity and the processes of establishing appropriate axonal connections are not well understood. Nevertheless, some evidence suggests that during development, differential cell adhesion may play a role in ganglia organisation; neurons and non-neuronal cells in ganglia have different levels of NCAM and levels of NCAM on the surface of cells correlated with differential abilities to form cell aggregates in vitro [85]. Thus adhesion may influence both composition and organisation of ganglia. Moreover, some insights concerning ganglia formation can also be obtained from various gut motility disorders. In contrast to HSCR patients that have hindgut aganglionosis and megacolon, gut dysmotility has also been reported in patients where enteric ganglia are abnormally large in size and/or number of neurons (hyperganglionosis) or reduced in size (hypoganglionosis). Hyperganglionosis occurs either as ganglioneuromas associated with multiple endocrine neoplasia type 2B (MEN2B), a heri disorder due to M918T missense mutation in the RET gene [86], or as intestinal neuronal dysplasia (IND), a controversial, inconsistently described entity, characterised by features that include increased density of submucosal ganglia, increased numbers of ganglion cells per submucosal ganglion and/or ectopic placement of ganglia [87]. Mice with mutations in the homeobox gene Enx (Hox11L1) have been suggested as a model for IND since these animals have megacolon and increased numbers of large intestinal myenteric ganglion cells [88]. In contrast to hyperganglionosis, hypoganglionosis, another condition that is difficult to diagnose by suction biopsy, has been associated with intestinal pseudo-obstruction (reviewed in [89]). Although the molecular mechanisms causing hypoganglionosis are unclear, the smaller ganglia may result from failure of development of neuronal subclasses [90] or from gene dosage effects since Gdnf +/− and Ret +/− mice have hypoganglionosis [91, 92].
Regarding establishment of neuronal projections within the ENS, limited data reveal that early-generated neurons that transiently exhibit tyrosine hydroxylase (TH) immunoreactivity have long leading processes that project caudally and will eventually give rise to caudally projecting neurons that innervate the circular muscle or other myenteric neurons [93]. Further, analysis of single cells at mid-gestation stages has shown that neurites in general have strong caudally biased directionality [47]. These observations led to the suggestion that the same factors that guide migration of ENCCs in a rostral-to-caudal direction are influencing the direction of axonal outgrowth of this neuronal population. However, although ENCCs often migrate along neurites, ENCCs are also observed in advance of neurites [47], suggesting that the correlation isn’t prescriptive. Indeed, in mutants in which neuronal processes show abnormal directionality, ENCC migration occurs normally [94], suggesting that ENCC migration is not simply guided by neurites. Another possible explanation for the correlation between ENCC migration and neurite projection is that migrating ENCCs can influence neurite outgrowth. Yet, following transplantation of ENCCs into aneural hindgut, some neurons project orally, despite confronting anally migrating ENCCs. Interestingly, sacral NC-derived ENCCs migrate along nerve fibres of the pelvic ganglia [41], and their migration may be more strictly dependent upon association with nerve fibres [95]. Thus it is likely that the factors influencing ENCC migration and neurite outgrowth of vagal- and sacral-derived ENCCs are distinct.
In the zebrafish, a correlation has been made between the orientation of smooth muscle cells and the direction of axonal projections; as circular muscle cells begin to differentiate and elongate around the circumferential axis, ENS neurons begin to extend axons circumferentially around the gut [96]. Whether such a putative organiser role for smooth muscle cell exists similarly in other vertebrate species is currently unknown. Finally, although neurons are known to make axonal connections to target tissues and express synaptic proteins even at embryonic stages [97–99], it is unknown at what time point neurons are making functionally active synaptic connections, although the relevant electrophysiological analyses are beginning to be performed [77].
Development of Interstitial Cells of Cajal
ICC: Different Forms, Different Functions
Interstitial cells of Cajal (ICC) are small network-forming cells located within the gut muscle layers that were first described by the Spanish neuroanatomist Ramon Santiago y Cajal in the late 1800s. However, it has only been in the last two decades that great progress has been made in our understanding of the morphology and physiological roles of ICC . These advances have been primarily due to the discovery that ICC express c-kit, the proto-oncogene that encodes the receptor tyrosine kinase Kit, the ligand for which is stem cell factor (SCF), and that anti-Kit antibody specifically labels ICC [100]. Consequently, studies using anti-Kit antibody in gut from humans and laboratory animals have revealed a range of different ICC morphologies in different gut regions ([101]; for reviews see [102, 103]). To investigate the physiological role(s) of ICC, their development was disrupted using either injection of anti-Kit antibody into mice to block ICC formation or, genetically, using W mutant mice that have loss-of-function mutations in the c-kit gene or steel mutant mice that are deficient in the SCF ligand for Kit. Morphological analysis of anti-kit-injected mice, or W or steel mutants, revealed a lack of ICC within the myenteric plexus of the small intestine, and physiological studies demonstrated a lack of intestinal pacemaker activity in the same gut region [100, 104–106]. Thus these studies demonstrated that ICC associated with the myenteric plexus are necessary for pacing electrical slow wave activity and contractions within GI muscles.
In addition to the pacemaker role for ICC, a role for ICC in the mediation of neurotransmission, as originally proposed by Cajal, has seemed likely since long, thin intramuscular ICC are closely apposed to varicose nerve terminals and electrically coupled via gap junctions to neighbouring smooth muscle cells [107]. Analysis of stomach tissues from W mutant mice that are deficient in intramuscular ICC, but have normal patterns of enteric nerve fibres and smooth muscle cells, demonstrated a lack of nitric oxide-mediated neuroregulation of smooth muscle [107]. These, and more recent findings for other neurotransmitters , confirm that intramuscular ICC play a fundamental role in the reception and transduction of both inhibitory and excitatory enteric motor neurotransmission [108] and reviewed in [109, 110].
More recently, other classes of interstitial fibroblast-like cells , such as those expressing platelet-derived growth factor receptor α (PDGFRα), have been reported to be present in the tunica muscularis of the gut [111, 112]. These cells have also been shown to be involved in transducing inputs from enteric motor neurons, this adding to the complexity of the cell types and interactions involved in the generation of coordinated gut neuromuscular activity.
Embryological Origin of ICC
ICC are derived from the mesoderm. Lecoin et al., using quail-chick interspecies grafting to genetically label the vagal neural crest cell-derived precursors of the ENS, demonstrated that in chimeric embryos, the ENS cells were of quail (donor) origin, whereas ICC were of chick (host) origin and therefore belonged to the gut mesenchyme lineage and were not neural crest-derived [22]. These authors also cultured aneural chick gut on the chick chorioallantoic membrane and found that ICC developed in the absence of enteric neurons, thus concluding that ICC are of mesodermal origin and develop independently from the enteric neurons with which they subsequently form anatomical and functional relations. The same year, Young et al., also using gut explants, but in this case from the mouse, demonstrated that when aganglionic segments of large intestine were explanted under the renal capsule of adult mice, ICC but not neurons developed in these explants [113], again indicating that ICC do not arise from the neural crest.
In the human gut, Kit-positive ICC have been identified as early as week 9 of development, after the colonisation of the gut by NCC and following the differentiation of the circular muscle layer. Unlike these other cell types, ICC do not appear to mature in a rostrocaudal wave, as Kit immunoreactivity is more defined in the hindgut than in the midgut at week 9. ICC rapidly mature and, by week 11, Kit immunoreactivity is restricted to cells surrounding the myenteric ganglia [7] (Fig. 2.1), in a pattern that is more organised in the midgut than in the hindgut. Similar reports of ICC surrounding myenteric ganglia have been described in the human foetal small bowel [114, 115]. ICC development in the human therefore appears to lag behind that of the ENS by at least 3 weeks and slightly behind that of smooth muscle differentiation, as evidenced by αSMA immunoreactivity. A similar developmental lag for ICC has also been reported in mouse and zebrafish embryos [116–118], as ICC form after the gut has been colonised by neural precursors and after the development of αSMA immunopositive muscle.
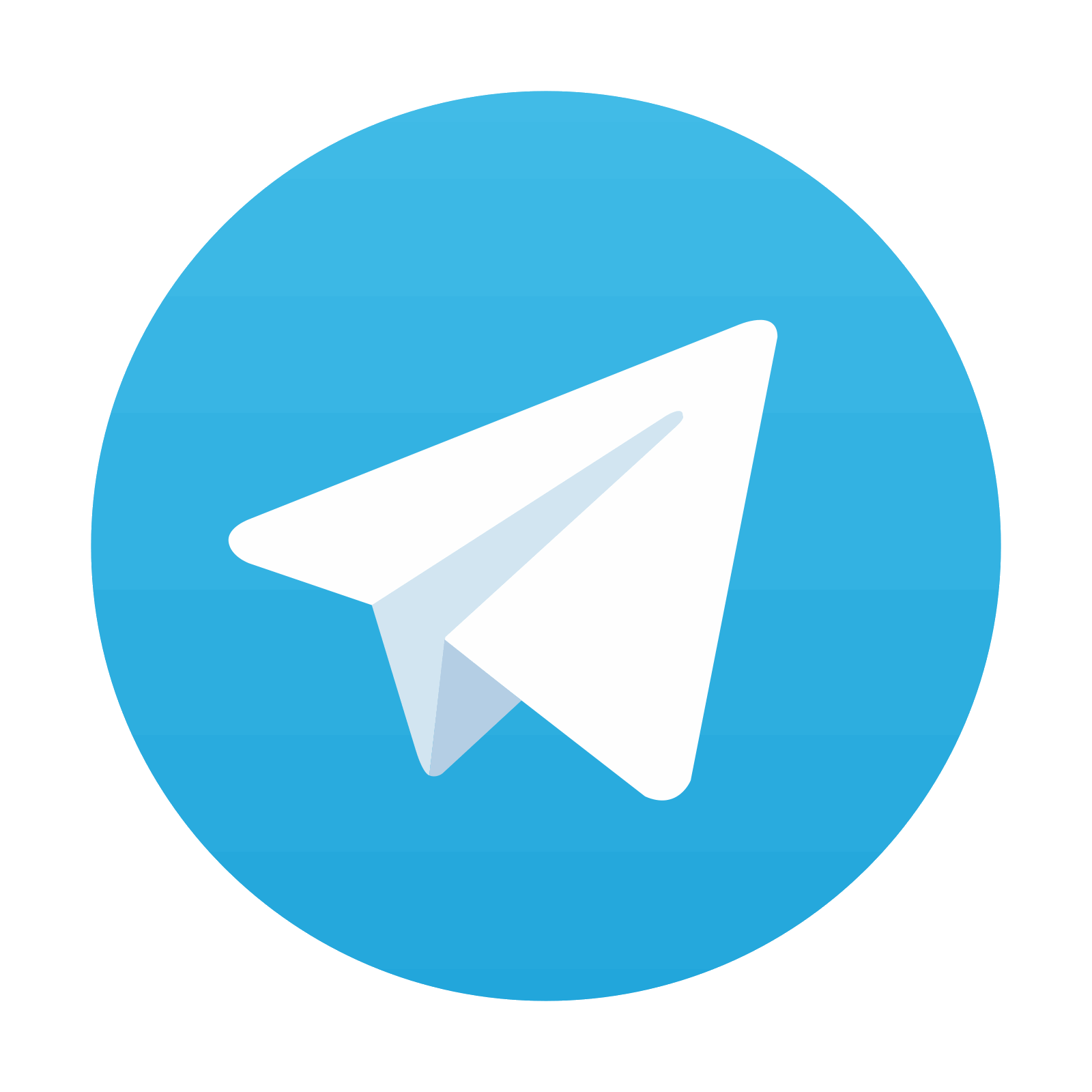
Stay updated, free articles. Join our Telegram channel
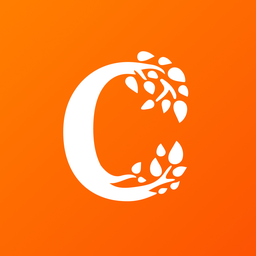
Full access? Get Clinical Tree
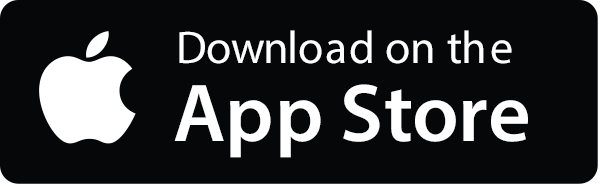
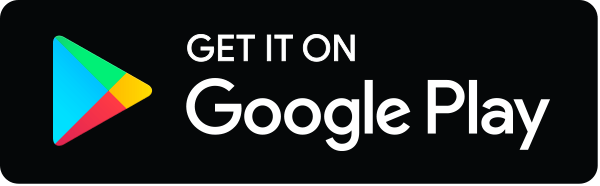