Abstract
Enteric neurons and glial cells arise from neural crest cells, most of which emigrate from the caudal hindbrain (“vagal” level) and then migrate into and along the developing gastrointestinal tract. There are also minor contributions from sacral neural crest cells and from Schwann cell precursors, which are associated with the axons of extrinsic neurons and give rise to some neurons after birth. The migration of enteric neural crest-derived cells is influenced by proliferation, cell-cell contact, neuronal differentiation, vascular endothelial cells, interactions with the extracellular matrix, dietary Vitamin A and by a large number of signaling pathways, the most important of which is the GDNF/RET signaling pathway. Different types of enteric neurons are born and develop at different ages, and some develop very early, shortly after neural crest-derived cells first enter the gut. Although propagating motility patterns are present 1 week prior to birth in mice, neurally mediated motility patterns are not present until around birth. Defects in the development of the enteric nervous system result in pediatric motility disorders, of which the best characterized is Hirschsprung’s disease.
Keywords
Neural crest, Enteric neuron, Migration, Differentiation, RET
Acknowledgments
LAS, MMH, and DFN are supported by NHMRC Australia grants 1079234, 1071153, and 1069757, respectively, and the research of TU and HE is supported by the Ministry of Education, Culture, Sports, Science, and Technology Science Research Funds Grant 2212005, Japan Society for the Promotion of Science Grant 25460279, and the Core Research for Evolutional Science and Technology, Japan Science and Technology Agency. We thank Annette Bergner, Lauren Young and John Stephenson for the images shown in Fig. 11.1 .
11.1
Origin, Migratory Pathways and Behavior of Enteric Nervous System (ENS) Precursors
The neural crest is a population of cells that emigrates from the dorsal neural tube around the time of neural tube closure. Neurons and glial cells of the enteric nervous system (ENS) arise from multiple sources of neural crest-derived cells.
11.1.1
Contribution of Postotic Hindbrain (Vagal) Neural Crest Cells to the ENS
Surgical removal of premigratory neural crest cells from different levels of the neural axis of chick embryos and chick-quail chimera studies showed that the most important source of enteric neurons is “vagal” neural crest cells, which emigrate from the caudal hindbrain adjacent to somites 1–7. Vagal neural crest cells enter the foregut ( Fig. 11.1 ), and then migrate caudally within the gut mesenchyme ( Fig. 11.2 ). In mice, when vagal neural crest-derived cells enter the midgut, the midgut and hindgut are transiently closely apposed, and a subpopulation of cells leaves the midgut, takes a short-cut through the mesentery and enters the colon without passing through the caecum. These “transmesenteric” neural crest-derived cells are then the front-runners to colonize the colon.


In human embryos, it takes around 3 weeks from when vagal enteric neural crest-derived cells (ENCCs) enter the foregut until they reach the anal end of the gastrointestinal tract , and in mice and chicks it takes 5 days.
11.1.2
Contribution of Sacral Neural Crest Cells to the ENS
Sacral level neural crest cells have been shown to give rise to some enteric neurons in mice, chick and quail. In mice, around 12% of all neural crest-derived cells in the distal hindgut are of sacral origin, while in the chick, sacral cells give rise to around 17% of neurons.
Studies in chick have shown that following surgical ablation of vagal neural crest cells, sacral crest cells cannot compensate for their loss, probably due to lower levels of expression of the receptor tyrosine kinase, RET, by sacral crest cells. In both mouse and chick, sacral neural crest cells aggregrate in the connective tissue adjacent to the hindgut, but then undergo a 2–3 day waiting period before they enter the hindgut ( Fig. 11.2 B). Although sacral neural crest cells do not enter the hindgut until after the hindgut has been colonized by vagal neural crest-derived cells, sacral crest cells do not require the presence of vagal neural crest cells to enter the hindgut, and the delay in the entry of sacral cells is due to the expression of inhibitory cues in the hindgut mesenchyme (see Section 11.2.10 ).
11.1.3
Contribution of Schwann Cell Precursors to the ENS
Sensory, visceromotor, and sympathetic neurons project their nerve fibers to the gut after the arrival of vagal ENCCs. Neural crest-derived Schwann cell precursors (SCPs) migrate along these extrinsic nerves (mesenteric nerves) and invade the gut. SCPs are derived from neural crest cells at all axial levels and associate with all nerves that project to peripheral tissues. Most SCPs in the mesenteric nerves of rodents differentiate into non-myelin-forming Schwann cells, which form bundles by surrounding several axons, and ensheath each axon in a pocket of cytoplasm without forming compact myelin. In contrast, enteric glial cells display distinct morphological features: (1) process extension over nerve fibers, (2) a partial sheath to nerve fibers, (3) long laminar processes between nerve processes and wrapping multi-axonal bundles. A fate-tracing study of SCPs revealed that a subset of SCPs generate enteric neurons postnatally, and contribute to a small proportion (< 5%) of submucosal neurons in the small intestine and < 20% of both myenteric and submucosal neurons in the large intestine. Given that SCPs are derived from all neural axial levels, SCP-derived enteric neurogenesis suggests the contribution of trunk neural crest to enteric neurons.
11.1.4
Hirschsprung’s Disease
The embryonic gut grows considerably in length while it is being colonized by ENCCs. For example, in mice, the post-caecal gut increases in length about fivefold between when ENCC first enter the post-caecal gut and when ENCC reach the anal end of the gut at E14.5. ENCC therefore migrate further than any other neural crest cell population. Failure of ENCC to colonize the entire length of the gastrointestinal tract results in distal aganglionosis, which in humans is called Hirschsprung’s disease. Because the ENS is essential for propulsive gut motility, infants with Hirschsprung’s disease suffer from intractable constipation and develop a megacolon, which is treated by surgical removal of the affected bowel region.
11.1.5
Migratory Behavior of Enteric Neural Crest-Derived Cells
After emigrating from the hindbrain neural tube, vagal neural crest cells migrate through paraxial tissues to the foregut. Retinoic acid is produced by paraxial tissues and induces the vagal cells to migrate in chains within the gut mesenchyme. ENCC chains migrate in close association with the neurites of caudally projecting, early enteric neurons.
While some vagal ENCCs must advance caudally towards the distal hindgut, a subpopulation of ENCCs must remain behind in each location to ensure that there are neurons present along the entire bowel. It had been assumed that the ENCCs that get left behind in each gut region had ceased migrating, however, time-lapse imaging studies have shown that the cells that remain behind continue to migrate for at least 24 h, but they migrate circumferentially, rather than caudally.
Sacral neural crest cells enter the hindgut along the axons of extrinsic pelvic plexus neurons and then migrate rostrally within the gut. Schwann cell precursors also enter the gut along the axons of extrinsic neurons.
11.2
Cellular and Molecular Regulation of ENS Development
The development of the ENS from neural crest-derived cells occurs as a series of overlapping processes including migration, proliferation, differentiation (neuronal and glial), neurotransmitter specification, axon growth and navigation, target selection, synapse formation and development of mature electrical properties. Importantly, migration, proliferation and neurogenesis occur concurrently and are linked (see Sections 11.2.1 and 11.2.2 ). A large number of molecular and cellular mechanisms have been shown to influence ENS development, but most of the mechanisms identified to date regulate the earlier events in ENS development-migration, survival, proliferation and neuronal differentiation-and little is known about the later events including neurotransmitter specification and synaptogenesis. The molecules involved in the development of the ENS are summarized in Table 11.1 , and the roles of some of these molecules and processes are also discussed in greater detail below and in several reviews.
Role | References | |
---|---|---|
Molecules required by vagal neural crest cells before entering the gut | ||
Geminin | Promotes survival of vagal NCCs by protecting against DNA damage | |
Retinoic acid | Regulation of Ret expression; migration, chain-formation and foregut colonization | |
Treacle (encoded by Tcof1 gene) | Survival of vagal NCCs | |
Molecules expressed by gut mesenchyme or epithelium | ||
GDNF | Survival, proliferation, differentiation and migration of ENCCs | |
Endothelin-3 | Proliferation of undifferentiated ENCCs, inhibits neuronal differentiation, promotes migration | |
NRTN (Neurturin) | Proliferation of undifferentiated ENCCs, promotes axon projections from excitatory motor neurons | |
Neurotrophin-3 | Survival and differentiation of developing enteric neurons | |
Sonic hedgehog | Proliferation of ENCCs, indirectly influence concentric patterning of neurons | |
Gli3 | Migration of ENCCs | |
Indian hedgehog | Survival of a subpopulation of ENCCs | |
BMP2, BMP4 (bone morphogenetic proteins 2 and 4) | Influence ENCC migration and differentiation, ganglion formation, concentric patterning of neurons | |
Netrin | Induce migration of subpopulation of ENCCs from myenteric region to submucosal region | |
Semaphorin 3A | Negatively regulates the time of entry of extrinsic axons and sacral neural crest cells into the distal hindgut | |
Retinaldehye dehydrognenases | Influence differentiation of ENCCs | |
Heparin-binding EGF-like growth factor (HB-EGF) | Migration of ENCCs | |
Molecules expressed by ENCCs (i) transcription factors | ||
SOX10 | Regulates Ret , Phox2b and Ednrb ; survival of ENCCs and development of glia | |
FOXD3 | Regulates Sox10 expression; maintains progenitors | |
PHOX2B | Survival of ENCCs by regulating RET expression | |
HAND2 | Terminal differentiation of subpopulation of enteric neurons | |
ASCL1 (MASH1) | Survival of regional subpopulation of ENCCs (esophageal ENCCs) and in the development of some enteric neuron subtypes in the intestine | |
PAX3 | Acts with SOX10 to activate Ret expression | |
AP-2 family | In zebrafish, ap2α acts with foxd3 to regulate Sox10 expression | |
ZEB2 (also called ZFHX1B or SIP1) | Specification of vagal neural crest cells. In cooperation with SOX10, regulates EDNRB expression | |
HLX | Required for migration of ENCCs beyond stomach | |
NR2F1 | Overexpression causes premature glial differentiation of ENCCs | |
TashT | Regulates ENCC migration speed | |
Molecules expressed by ENCCs (ii) cell surface receptors | ||
RET | Signaling molecule for GDNF and NRTN | |
GFRα1 | GPI-anchored receptor to which GDNF binds | |
GFRα2 | GPI-anchored receptor to which NRTN binds | |
Ednrb | Receptor for endothelin-3 | |
Ntrk3 (also called TrkC) | Receptor for neurotrophin-3 | |
ErbB3 | Development of enteric glia | |
Molecules expressed by ENCCs (iii) other | ||
Spry2 (also called Sprouty homolog 2) | Negative regulator of RET | |
KIF26A | Negative regulator of RET | |
L1-CAM | Influences ENCC migration and rate of neuronal differentiation | |
NCAM | Influences clustering of developing enteric neurons | |
β1-integrin (Itgb1) | Rregulates ENCC migration, ganglion geometry | |
C3a and cadherin | Regulates ENCC cell adhesion and directionality of migration | |
collagen VI | Influences ENCC migration speed | |
tenascin-C | Influences ENCC migration | |
Inosine 5′ monophosphate dehydrogenase 2 (IMPDH2) | Proliferation of vagal NCCs | |
Notch | Regulates progenitor proliferation and glial development | |
Kif1-binding protein | Promotes neurite formation | |
Pten | Inhibits ENCC migration and proliferation | |
Phactr4 | Influence directionality of ENCC migration via regulation of integrin signaling | |
small GTPases | Regulates ENCC migration, proliferation and neurite extension | |
Neuregulin | Survival of postnatal enteric neurons | |
microRNAs | Neuronal differentiation | |
Electrical activity/neurotransmitters | ||
Sodium-dependent action potentials | Regulates rate of neuronal differentiation of some neuron subtypes | |
Serotonin | Regulates neuronal differentiation | |
Slc6a2 (also called NET, norepinephrine transporter) | Survival and/or differentiation of some subtypes of enteric neurons | |
Nitric oxide | Influences ENCC differentiaton and motility in chick embryos | |
Environmental factors | ||
Microbiota | Signals from the microbiota regulate the initial colonization and homeostasis of the mucosal enteric glia | |
Diet: Vitamin A/retinoic acid | Promotes ENCC migration | |
Medicine: ibuprofen | Reduces speed of ENCC migration | |
Medicine: mycophenolate | Impairs ENCC proliferation and migration |
11.2.1
Proliferation/Cell Number Is Essential for Migration
There are more neurons in the ENS than there are in the spinal cord. The many millions of enteric neurons and glial cells arise from a small population of neural crest-derived cells (estimated to be around 1000 cells) that enter the foregut. Hence, immense proliferation of ENCCs must occur during development of the ENS.
ENCC number plays a vital role in the colonization of the gut. For example, surgical reduction of the number of vagal neural crest cells in chick embryos reduces the distance that ENCCs migrate along the gut and therefore results in aganglionosis of the most distal regions of the gut, similar to Hirschsprung’s disease. Proliferation of ENCCs is essential both en route to the gut and while migrating within the gut. In contrast to neural crest-derived cells in sympathetic and dorsal root ganglia that undergo a short, but rapid, proliferative period, many ENCC remain proliferative throughout embryonic development in mice. The role of cell death in ENS development is discussed later in this chapter ( Section 11.2.6 ).
11.2.2
Effects of Neuronal Differentiation on ENCC Migration
While ENCCs are migrating along the developing gut, a subpopulation starts to differentiate into neurons and expresses pan-neuronal markers. Inhibition of endothelin receptor B (EDNRB) signaling results in both premature neuronal differentiation and delayed migration of ENCCs (see Section 11.2.8 ), which lead to the idea that early or excessive neuronal differentiation of ENCCs directly or indirectly retards their migration. ENCCs expressing pan-neuronal markers continue to migrate, but at a slower rate than the ENCC population. Neuronal differentiation is likely to retard ENCC migration indirectly by reducing the number of proliferating progenitors. However, neuronal differentiation might also promote the colonization of the gut by ENCCs, as time-lapse imaging studies have shown that ENCCs use neurites as a migratory substrate.
11.2.3
Cell/Cell Contact Between ENCCs Influences Migration
Time-lapse imaging studies have shown that ENCCs migrate mostly in chains. A key role for cell-cell contact in ENCC migration is suggested by studies that have shown that reducing ENCC number retards ENCC migration (see Section 11.2.1 ). Inhibition of L1CAM (L1 cell adhesion molecule) signaling or complement anaphylatoxin C3a signaling in gut explants in vitro increases the number of solitary ENCCs and retards the rate of migration. Moreover, when a small number of the most caudal ENCCs were surgically isolated from other ENCCs, they migrated slower, reinforcing the notion that cell-cell contact is important for ENCC migration.
11.2.4
Role of Vascularization in ENCC Migration
In chick embryos and zebrafish larvae, ENCCs migrate in close association with endothelial cells, which already exist as a network in the gut wall. Furthermore, when endothelial cell development is pharmacologically inhibited in chick embryos, ENCC migration is perturbed, suggesting that blood vessels act as a substrate to promote ENCC migration. However, while there is a temporal and spatial correlation between ENCC migration and primary vascularization in the embryonic mouse gut, ENCC migration is unaffected when gut vascular development is genetically impaired. Thus, there might be species differences in the role of vascularization in ENS development.
11.2.5
The Extracellular Matrix (ECM) Influences ENCC Migration
Numerous ECM molecules are present within the developing gut. The principle ECM receptors for ECM molecules are integrins. Neural crest-specific knock out of the β1-integrin, a monomer common to most ECM receptors at embryonic stages, results in slowed and incomplete colonisation of the hindgut in the mouse. In addition, even in the region that is colonised by ENCC, the ganglion size, shape, and geometry are perturbed. This points strongly to the importance of interactions between ENCCs and the ECM in establishing the normal ENS.
A variety of ECM molecules have been shown to influence ENCC migration, and some of these ECM components are produced by the ENCCs themselves. The ECM glycoprotein, tenascin-C, promotes ENCC migration and is produced by both ENCCs as well as other cell types in the developing gut. Collagen VI is also produced by ENCC, but excess collagen VI production is inhibitory to ENCC migration. Thus, production of the correct amounts of ECM molecules by ENCCs and other cell types is essential for normal ENCC migration.
11.2.6
Apoptosis in ENS Development
In some parts of the developing nervous system, up to 50% of young neurons die. Although perturbations to RET signaling result in dramatic increases in cell death in the developing ENS, apoptosis was originally thought to be a relatively rare event during normal ENS development. Later studies demonstrated apoptosis in vagal neural crest cells in chick embryos prior to their entry into the gut, and showed that inhibition of caspase-9 in vagal crest cells resulted in hyperganglionosis. Thus apoptosis does appear to play a role early in normal ENS development by regulating the number of ENS progenitors prior to their entry into the gut.
11.2.7
GDNF Family Ligands/GFRα/RET Signaling
GFLs (GDNF Family Ligands) are a neurotrophic factor family that includes GDNF, neurturin (NRTN), artemin (ARTN), and persephin (PSPN). GFLs bind to the GDNF family receptor alphas (GFRαs), which are GPI-anchored cell surface proteins, and activate the RET tyrosine kinase.
GDNF is expressed in the gut mesenchyme whereas RET and GFRα1 are expressed in immature ENCCs as early as they invade the gut wall. Gdnf -, Gfra 1-null, and Ret -null mice lack enteric ganglia in the gut below the stomach. This phenotype is already evident soon after ENCCs enter the foregut and is likely to be caused by compound deficits in migration, proliferation and survival of ENCCs. Conditional inactivation of RET or GFRα1 after gut colonization by ENCCs causes massive death of ENCCs specifically in the colon, suggesting that GDNF signaling via RET/GFRα1 is essential for the survival of colonic ENCCs.
RET deficiency also affects expression of neuronal nitric oxide synthase (nNOS), which catalyzes the production of nitric oxide, a neurotransmitter involved in the relaxation of gastrointestinal smooth muscle. The exact ligand and GFRα receptor involved in nNOS expression are unclear.
In culture, GDNF acts not only as a neuronal survival factor, but also as a mitogen for immature ENCCs. This mitogenic activity is thought to be a crucial driving force for ENCCs to undergo caudal migration as a whole (see Section 11.2.1 ). In addition to promoting ENCC migration indirectly by promoting proliferation, GDNF probably also promotes the migration of ENCCs directly. .
ENS development is sensitive to the levels of RET signaling. In humans, RET haploinsufficiency can result in Hirschsprung’s disease, while homozygous RET mutations have been associated with total intestinal aganglionosis. In mice, a reduction in Ret gene expression to around one-third of normal levels impairs migration and survival of ENCCs. Gdnf +/− mice have hypoganglionosis (a reduction in around 50% in the number of enteric neurons) and abnormal motility, whereas mice with augmented GDNF availability to the ENS have increased numbers of enteric neurons. Negative regulators of RET signaling are also important in the developing ENS. Mice lacking Kinesin superfamily protein 26A (KIF26A) or the tyrosine kinase inhibitor, Sprouty2, have hyperganglionosis (an increase of around 50% in the number of enteric neurons) and motility disorders due to overactivated GDNF-RET signaling. Thus, both under- and overactivation of the RET signaling pathway result in defects in ENS development and consequent motilty disorders.
In summary, GDNF signaling via RET/GFRα receptors is pleiotropic in ENS development, regulating multiple vital processes including migration, proliferation, survival, and differentiation of ENCCs.
NRTN signaling via GFRα2 plays a more limited role in ENS development. The ENS is formed in adult Nrtn – and Gfr α 2 -null mice, but myenteric neurons exhibit reduced cell soma size and fewer excitatory nerve fibers are observed in the myenteric plexus. Nrtn -null mice also display a partial loss of submucosal neurons.
11.2.8
Endothelin-3/EDNRB (Endothelin Receptor Type B) Signaling
Endothelin-3 is produced by the mesenchyme of the developing gut, with the highest expression in the caecum. . Endothelin-3 activates a G protein-coupled molecule, EDNRB, which is expressed by neural crest-derived cells as well as the gut mesenchyme. Mice, rats, and horses that lack EDNRB or endothelin-3, and humans with mutations in the genes encoding these molecules, lack enteric neurons in the most distal regions of the bowel. Endothelin-3 appears to promote the migration of ENCCs both directly, and also indirectly by promoting proliferation and inhibiting neuronal differentation. One mechanism by which endothelin-3 influences ENCC migration is by stimulating cell adhesion. In mice lacking EDNRB, the delay in migration of ENCCs is exacerbated by changes in the hindgut microenvironment with developmental age that make it less permissive to ENCC migration.
11.2.9
Transcriptional Regulation of ENS Development
A network of transcription factors, some of which are common to all peripheral autonomic neurons, regulates ENS development. Here, we briefly discuss the roles of SOX10 and PHOX2b. Other transcription factors that have also been shown to play a role in ENS development are listed in Table 11.1 .
Sox10 is expressed in all premigratory neural crest cells where it plays a key role in inducing migration. SOX10 is also a pivotal transcriptional regulator of ENS development because it is necessary for the induction of both Ret and Phox2b and because it regulates Ednrb expression in cooperation with another transcription factor, ZEB2. Loss of SOX10 in mice results in the death of vagal neural crest cells prior to their entry into the gut. SOX10 is also required at later stages of ENS development for the differentiation of enteric glial cells.
Like Sox10 null mutants, mice lacking PHOX2B do not have enteric neurons in any region of the gastrointestinal tract. There is no RET expression in ENCCs in Phox2b −/− mice and hence PHOX2B plays a key role in ENS development because it is required for Ret expression. PHOX2B can also repress Sox10 , which might be important for neuronal differentiation.
11.2.10
Axon/Neuronal Guidance Molecules—Slit, Netrins, Semaphorin 3A
Although some Schwann cell precursors derived from trunk neural crest give rise to enteric neurons during late embryonic development (see Section 11.1.3 ), trunk level neural crest cells do not enter the gut immediately after emigrating from the neural tube. Slits are expressed in the splanchnic mesoderm dorsal to the gut, and Robo receptors are expressed by trunk neural crest cells. It has been proposed that Slit-Robo signaling prevents trunk neural crest cells from entering the gut. However, even when cocultured in direct apposition with explants of embryonic gut, trunk neural crest cells give rise to few enteric neurons, indicating that Slit-Robo signaling is not the only mechanism precluding trunk neural crest cells from colonizing the gut after leaving the neural tube.
In mammals, the first enteric ganglia to develop are in the myenteric region, immediately outside of where the circular muscle layer forms. Submucosal ganglia form later following the centripetal migration of a subpopulation of ENCCs. Netrin is expressed by gut epithelial cells, and its receptor, deleted in colonic cancer (DCC), is expressed by ENCCs. Netrin appears to act as a chemoattractant to induce the centripetal migration of neurons to form submucosal ganglia, as submucosal ganglia do not form in DCC null mutant mice.
Sacral neural crest cells undergo a waiting period prior to entering the distal hindgut (see Section 11.1.2 ). In both chick and mouse embryos, Semaphorin 3A is initially expressed by all mesenchymal of the distal hindgut. The outer mesenchymal cells subsequently downregulate Semaphorin 3A expression, which coincides with the entry of sacral neural crest cells into the hindgut. There is premature entry of extrinsic axons and sacral neural crest cells into the hindgut of mice lacking Semaphorin 3A, confirming that Semaphorin 3A acts as an inhibitory cue to regulate entry of extrinsic axons and sacral neural crest cells into the gut.
11.2.11
Cell Adhesion Molecules—L1CAM
L1CAM is expressed by many ENCCs. Perturbing L1CAM activity retards the migration of ENCCs along gut explants, and there is also a delay in the migration of ENCCs along the gut of L1cam null mutant mice, although the entire length of the gut is eventually colonized. Human infants with mutations in L1CAM have severe CNS defects, and some also have Hirschsprung’s disease. Studies using gut explants have shown that L1CAM is probably important for ENCC migration because it promotes cell-cell contact between ENCCs, particularly close to the migratory wavefront.
11.2.12
Dietary Factors—Vitamin A and Medicines
Retinoic acid, a metabolite of Vitamin A, influences the proliferation and differentiation of ENCCs in vitro. Mice lacking serum retinol-binding-protein (Rbp4), which is required for the transport of hepatic retinol to peripheral tissues, can be depleted of peripheral tissue retinoids by eliminating vitamin A from the diet. Vitamin A depletion of pregnant female Rbp4 −/− mice at a stage prior to the entry of ENCCs into the gut of their embryos resulted in a delay in ENCC migration and distal aganglionosis.
The commonly used anti-inflammatory medicine, ibuprofen, reduces the speed of ENCC migration in zebrafish and chick embryos, and in mice genetically predisposed to Hirschsprung disease. Mycophenolate, an immunosuppressant, has also been shown to inhibit ENCC migration in zebrafish and mice. Thus, dietary factors and some drugs might increase the risk of Hirschsprung disease in genetically susceptible infants.
11.2.13
Interactions Between Signaling Pathways
ENS development can be influenced by interactions between signaling pathways. Interactions between RET and EDNRB were first revealed in studies of humans with Hirschsprung’s disease. . Studies in mice verified that interactions between RET and EDNRB influence the severity and penetrance of aganglionosis. . Subsequent studies have reported additional interactions that can influence ENS development; between RET and endothelin-3, SOX10 and EDNRB, SOX10 and SOX8, SOX10 and endothelin-3, SOX10 and L1CAM, EDNRB and L1CAM, endothelin-3 and L1CAM, SOX10 and β1-integrins, PAX3 and CDX, N-cadherin and β 1-integrins, endothelin-3 and β 1-integrins, and SOX10 and ZFHX1b. Some of the interactions are direct, whereas others are indirect. For example, Sox10 directly regulates the expression of Ednrb and L1cam , whereas at least some of the interactions between the RET- and EDNRB-signaling pathways are likely to be due to common downstream signaling molecules, such as protein kinase A.
11.3
Development of Enteric Neuron Subtypes, Glial Cells, and Ganglia
In embryonic mice, a subpopulation of ENCCs starts to express pan-neuronal markers at E10.5, which is shortly after ENCCs first enter the gut (see Section 11.2.2 ). In the adult mouse, around 10 subtypes of myenteric neurons with different functions have been identified. ENCCs expressing two enteric subtype markers—nNOS and calbindin—are present at E11.5. Other enteric neuron subtypes are first detected at later developmental stages, and some do not appear until after birth. Different enteric neuron subtypes also exit the cell cycle at different, but overlapping, ages.
Little is known about the mechanisms controlling the development of different neuron subtypes in the gut. The transcription factor, ASCL1, regulates the development of multiple enteric neuron subtypes, the development of nNOS neurons is senstive to the levels of RET signalling and NRTN/GFRα2/RET signalling plays a role in the development of substance P and cholinergic neurons (see Section 11.2.7 ).
There is now strong evidence that early differentiating enteric neurons influence the differentiation of undifferentiated ENCCs. Enteric neurons are one of the earliest populations of neurons in the nervous system to exhibit electrical activity. At E11.5, many different voltage-dependent ion channels are expressed in the ENS and a subpopulation of enteric neurons can fire action potentials. Neural activity influences the differentiation of some subtypes of enteric neuron. Moreover, mice lacking the norephinephrine transporter (NET) or Tph2, the synthetic enzyme for serotonin, have defects in the ENS and it appears that synthesis and release of neurotransmitters from neurons generated early in ENS development is important for the differentiation or survival of neuronal subtypes that are generated later in ENS development.
The first enteric neurons to develop have neurites that project caudally along the same pathway as ENCCs migrate ( Fig. 11.2 C), but the mechanisms that control axon navigation and that link axon projection with synthesis of the appropriate neurotransmitter are unknown.
Enteric ganglia contain many more glial cells than neurons. SOX10 is essential for early ENS development because it is required for RET and Phox2b expression (see above). Sox10 is also required later in ENS development for the differentiation of glial cells (see Section 11.2.8 ). Bone morphogenetic proteins (BMPs), the transmembrane receptor, Notch, the secreted molecule, glial growth factor 2, and the receptor, ErbB3, have been shown to be involved in the development of enteric glia.
Enteric neurons are clustered into ganglia. Individual ganglia are not clonal, but form by the clustering of neurons and glia cells. Loss of β1-integrin expression from ENCCs in vivo results in both retarded ENCC migration and abnormal aggregations of ENCCs, but it is unclear whether the abnormal clusters are an indirect effect of the defective migration, or whether β1-integrin normally plays a role in regulating the size of ganglia. Studies in vitro using explants of embryonic gut or immunoselected ENCCs have shown that exogenous BMPs enhance, and blocking BMP signaling inhibits, aggregation of ENCCs. The effects of BMPs on ENCC aggregation appear to be mediated by polysialation of neural cell adhesion molecule (NCAM1). It has been proposed that the morphology of enteric ganglia is controlled by differential adhesion of neurons and nonneuronal ENCCs, with neurons having higher adhesion than nonneuronal ENCC.
Although some neurons develop early during ENS development (see above), studies in mice have shown that there are also significant changes to the ENS during postnatal development including the generation of additional neurons from Schwann cell precursors (see Section 11.1.3 ), morphological, and electrophysiological changes in some subtypes of neurons and changes in neurotransmitter receptors. Furthermore, after birth, the gut microbiota regulates the colonization of the lamina propria by glial cells.
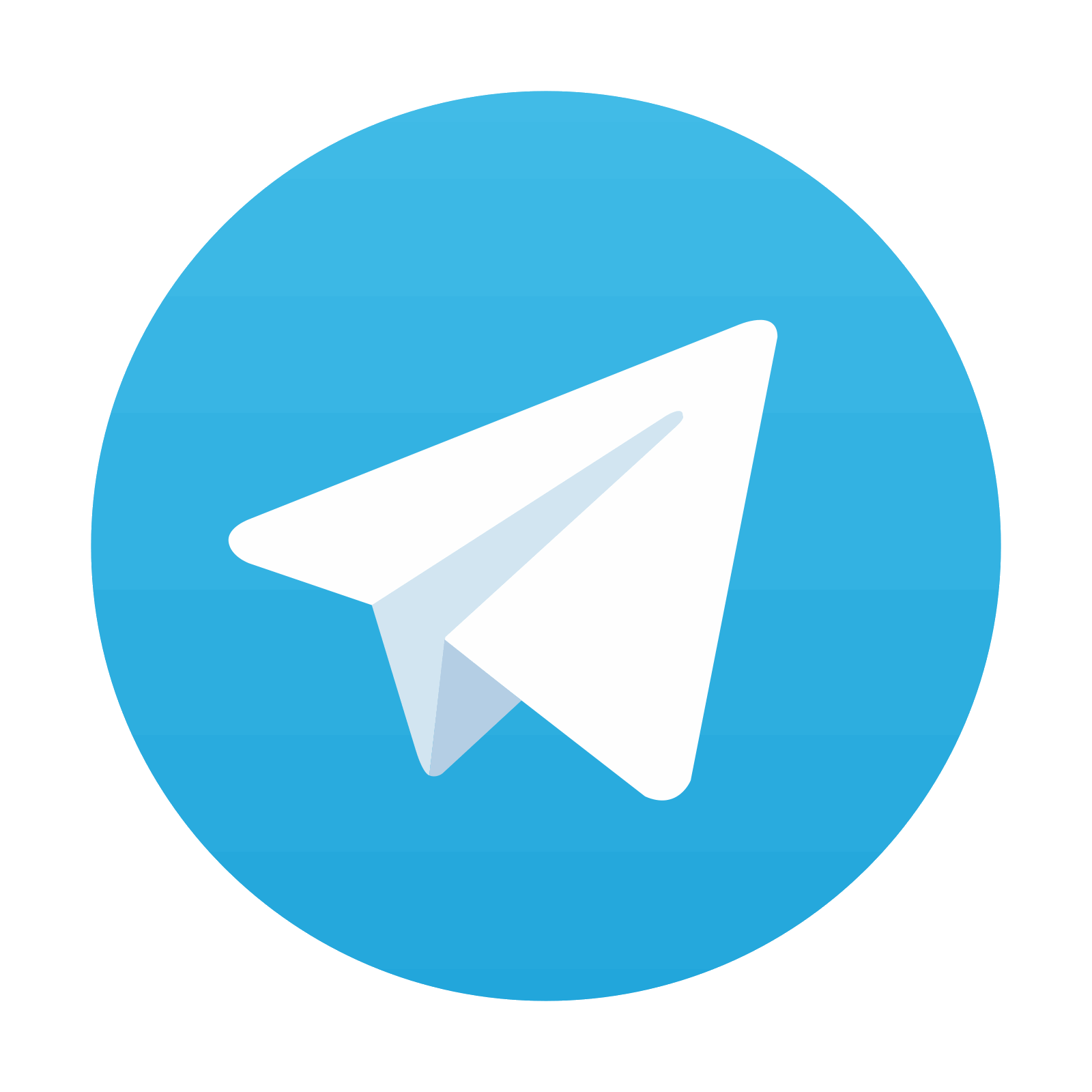
Stay updated, free articles. Join our Telegram channel
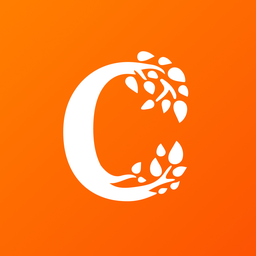
Full access? Get Clinical Tree
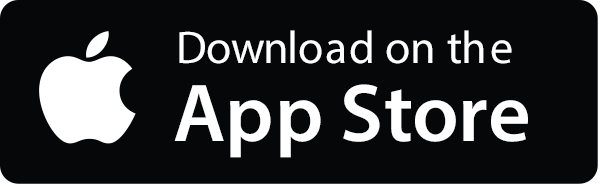
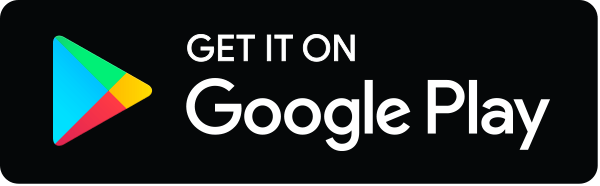