Fig. 5.1
Normal erythropoiesis. The hematopoietic stem cell (HSC), once committed, differentiates into the blast-forming units—erythroid (BFU-E) that in turn differentiates into the colony-forming unit—erythroid (CFU-E). The proerythroblast (Pro-EB), the next stage, is the last nonspecific progenitor cell. This then goes through multiple stages of maturation through the basophilic (Baso EB), polychromatophilic (Poly EB), and orthochromatic (Ortho EB) erythroblasts before losing the nucleus during the late normoblast (Late NB) stage. Then the cell is extruded into the circulation as a reticulocyte (Ret) which then becomes the erythrocyte (Ery). EPO-dependent stages include CFU-E until the Baso EB stage. The entire cycle takes about 7 days

Fig. 5.2
Pathologic erythropoiesis. Normal erythropoiesis leads to less than half survival rate of the basophilic erythroblasts to eventually become erythrocytes (Ery). Under low EPO states, the EPO-dependent cells undergo apoptosis and hence less number of Ery are produced leading to anemia. Giving rHuEPO (green arrow) restores erythropoiesis to normalcy provided iron is adequate. The cells themselves are usually normocytic. In certain vitamin deficiencies, there is inefficient erythropoiesis and hence macrocytes are produced. In hemorrhagic or hemolytic states, EPO levels are higher and this leads to an increased Ery production. In iron deficiency with elevated EPO, though the EPO-dependent cells’ production is enhanced, during hemoglobin synthesis the inhibited protein synthesis results in microcytes and a lower number of Ery
Erythropoietin
Erythropoietin (EPO) was identified as a vital cog in erythropoiesis in the early 1940s and named “erythropoietin” in 1948 [1]. EPO is produced by cortical peritubular fibroblasts located near the proximal tubular cells in the outer medulla and inner cortex in the kidney [2]. This production is expanded into the outer cortex in response to hypoxia and anemia, a region that is especially susceptible to hypoxia [3]. Indeed, tissue hypoxia is the pivotal factor that increases EPO production as a final step in a signal transduction pathway involving several proteins among which hypoxia-inducible factors (HIFs) play a central role.
Though EPO transcripts have been isolated from several other tissues, they do not contribute significantly to circulating levels; 90% of all EPO produced in the body is produced in the kidneys and about 10% is produced in the liver [4, 5]. EPO receptors (EPO-R) bind circulating EPO and initiate signal transduction within the burst-forming unit-erythroid (BFU-E), the first cell in the erythropoietic cellular lineage to express the EPO-R [6]. The EPO-R exists as a homodimer bound to Janus Kinase-2 (JAK-2) protein and in turn to transferrin receptor 2 (TfR-2) [7]. Upon binding EPO, the EPO-R complex is internalized and undergoes conformational change leading to a cytoplasmic segment of the complex being phosphorylated and stimulating multiple signaling pathways within the cell leading to gene transcription [4, 8]. The effective half-life of EPO is only about 30–40 min because of dephosphorylation and degradation of both EPO-R and JAK-2 [9].
Due to the central role of the kidneys in EPO production, it is not surprising that anemia is a nearly universal complication with progressive loss of glomerular filtration rate (GFR) , developing gradually and increasing in severity as kidney disease progresses [10]. Indeed, anemia becomes a ubiquitous problem by the time patients are nearing the need for renal replacement therapies [10, 11]. The most common reason for the anemia characteristic of chronic kidney disease (CKD) is a relative insufficiency of EPO production [11], although the complex clinical picture of most patients suffering from CKD often includes additional conditions complicating the etiology of anemia, such as inflammation or iron deficiency. Nevertheless, pharmacologic replacement of EPO has revolutionized the approach to anemia in patients with CKD. Until the 1980s, anemia of CKD was managed with regular blood transfusions and obviously, this came with several complications such as sensitization, transfusion reactions, secondary iron overload, etc. [12]. This resulted in a generally accepted trend of lower hemoglobin levels in end-stage renal disease (ESRD) patients. Since its introduction, EPO and newer synthetic forms have formed the cornerstone of anemia management in CKD.
Recombinant Human Erythropoietin and Its Derivatives: Clinical Considerations
Recombinant human erythropoietin (rHuEPO) was developed in the 1980s and was noted to have a surprisingly high efficacy in correcting anemia of CKD [13] (Table 5.1). The extraordinary success of rHuEPO led to the spawning of several derivatives of the original molecule that can be collectively called epoetins or erythropoiesis-stimulating agents (ESAs). rHuEPO and its derivatives are glycosylated proteins and hence are produced from mammalian cell lines and in vivo action is dependent on the degree and nature of glycosylation. The epoetins α and β were the first molecules manufactured and became widely used after supportive evidence from multiple clinical trials. The typical dosing schedule of rHuEPO in routine clinical practice is three times a week, with the medication being administered along with the HD treatment, typically by the dialysis nurse. This schedule also assures a close to perfect adherence with the prescribed regimen, enhancing the effectiveness of the therapy.
Table 5.1
Currently available rHuEPO agents and other EPO-based agents in the US
Molecule | Brand name | Company(s) | Year introduced/approved | Major trials | Status | |
---|---|---|---|---|---|---|
1 | Epoetin-α | Epogen®, Eprex®, Erypo® | Amgen, Janssen | 1989 (Epogen in the US), 1990 (Eprex, Erypo in Europe) | Active | |
2 | Epoetin-β | NeoRecormon® | Boehringer-la Roche | 1990 (Europe) | Bennett [205] | Active |
3 | Darbepoetin-α | Aranesp® | Amgen | 2001 (US and Europe) | TREAT [60] | Active |
4 | Epoetin-δ | Dynepo® | Shire | 2005 (outside US) | Martin et al. [206] | Withdrawn |
5 | CERA | Mircera® | Roche | 2007 | Active | |
6 | Peginesatide | Omontys® | Affymax | 2012 | Emerald 1, 2 [82] Pearl 1, 2 [81] | Suspended/restricted [80] |
7 | HX575 (biosimilar) | Binocrit® (Europe) | Sandoz | 2007 (Europe), pending (US) | Monitor-CKD5 [207] | Active/pending |
8 | SB309 (biosimilar) | Retacrit® (Europe) | Hospira | 2007 (Europe), pending (US) | Phase 3 Trial [208] | Active/pending |
9 | Epoetin- α biosimilar | – | Amgen | Pending | SHADE [209] | Pending |
Intravenous (IV) administration continues to be the preferred route for rHuEPO in patients receiving maintenance HD, even though the bioavailability of the IV route is inferior compared to the subcutaneous (SQ) route [14]. The half-life of rHuEPO in vivo is only about 4–11 h [15]. SQ administration leads to a more sustained increase in serum rHuEPO levels; the bioavailability is 20–25% and measurable rHuEPO levels can be detected even 4 days after administration [15, 16]. The SQ dose is also ~25% lower for the same clinical effect compared to the IV dose, thus providing cost benefits [17, 18]. One complication possibly linked to SQ use of certain products is pure red cell aplasia (PRCA) [19]. As a result of this possibly idiosyncratic reaction, the SQ route has become less popular in spite of its multiple other benefits.
Following the successful adoption of rHuEPO, there have been several attempts to pharmacologically manipulate its structure in order to achieve a more favorable therapeutic profile. Darbepoetin-α was the first such agent introduced; due to enhanced glycosylation darbepoetin has a larger size and a much longer half-life compared to rHuEPO [20, 21]. In the case of darbepoetin the route of administration (IV vs. SQ) does not seem to influence efficacy, and less frequent dosing makes it ideal for certain patients such as those on home dialysis therapy. Adoption has been slow due to costs but has been favored once incorporated into center-specific protocols. A conversion factor of 200 units rHuEPO to 1 μg of darbepoetin is typically used for initial dosing. Another newer agent developed to further increase the half-life of rHuEPO was the continuous EPO receptor activator (CERA) , created by binding EPO to polyethylene glycol. CERA has a half-life of 5 days irrespective of route of administration [22, 23]. Studies have proven the non-inferiority of CERA [24], which has been used for many years now in other countries, and also recently started being adopted by dialysis providers in the US for anemia management. Introduction of CERA (Mircera®, Roche) into the market was supported by its non-inferiority vs. darbepoetin in a randomized trial in CKD patients [25]. With a very long half-life and extended administration intervals, CERA offered a convenient alternative in non-dialysis CKD [26] and peritoneal dialysis patients whose contact with the dialysis providers is not on a daily basis [27]. IV CERA was pitted against darbepoetin in dialysis patients in a randomized trial and was found to be clinically as effective in maintaining Hgb levels in the target range as darbepoetin [28], thus paving the way for its mainstream use. Though cost-effectiveness is still not proven [29], CERA is a promising addition to the quiver of agents that are currently at our disposal.
Biosimilars and “Copy” Epoetins
Medicines that mimic biologic molecules but are produced artificially, that are clinically similar in action but having minor differences in clinically inactive metabolites are called biosimilars. Biosimilar peptides have been around for several years now and have been successful when used in many different diseases and conditions. A wide variety of drugs are available in this class including hormones, vaccines, growth factors, monoclonal antibody-based agents, etc. In the strictest sense, the advent of biosimilars in the management of anemia occurred with the introduction of darbepoetin. That said, the term “biosimilar” is currently applied to the generic formulations of the patented molecules epoetins-α and β in the US. In Europe, the first few biosimilars licensed were generic manipulations of epoetins-α and ζ (zeta) after their patent expirations [30]. The complex structure of these proteins necessitates an equally complicated manufacturing process, which results in subtle differences in the end product of different manufacturers. These end products also differ in their pharmacologic actions [31]. Therefore, an identical replication of epoetins-α or ζ is not possible, even though their main biologic actions are compatible with the mechanism of action of rHuEPO; hence the term “biosimilar” is applied to them. Since they are produced in different cell lines, it is possible that there are isoforms in the same batch of products that can alter clinical pharmacology; possibly contributing to serious side effects such as PRCA [32, 33]. One deterrent to the manufacturing of biosimilars was the discovery of several cases of PRCA with one of the epoetin-α biosimilar brands [34]. Another was the ever-decreasing price of the novel ESAs and hence the loss of financial impetus to continue to make newer biosimilars. With patent expiration of the original ESAs, the introduction of several biosimilars of rHuEPO onto the US market is imminent.
In the study on the efficacy of biosimilars , the first trial compared the efficacy of the molecule HX575, the first biosimilar approved in Europe in 2007, to original rHuEPO molecules. Based on the structure of epoetin-α, HX575 was pharmacokinetically equivalent to epoetin-α but patient exposure was different as seen in the area under the curve (AUC) levels [35]. This finding is suggestive of different clinical response to equivalent dosing with biosimilars and hence close monitoring to hematologic indices was recommended [36]. When given to patients as maintenance therapy for anemia, HX575 was deemed clinically equivalent to epoetin-α [37] and as safe as epoetin-α [38]. However, with some evidence of differing clinical efficacy based on manufacturing locations [39], it was not deemed feasible to reliably incorporate HX575 in anemia protocols. SB309 was the second biosimilar agent to be developed based on epoetin-ζ. This molecule’s lower bioavailability compared to epoetin-α was attributed to the higher protein content of epoetin-α [40]. SB309 was found to be clinically equivalent to epoetin-α in the initial correction phase study and in two maintenance trials [41–43]. This was further corroborated in a crossover trial with a similar safety profile as epoetin-α [44]. Immunogenicity is a legitimate concern with biosimilars and this was also studied in ESA-naïve CKD patients. Of 337 patients randomized to receive either HX575 or SB309 via the SQ route, two patients developed antibodies and one had PRCA [45]; this was attributed to tungsten exposure during manufacturing [46]. None of the biosimilar studies have reported such antibodies when given IV [47]. Due to the rare nature of this adverse effect, only long-term clinical use will provide robust information about the magnitude of PRCA with biosimilars.
In the US, the first biosimilar agent for any indication was approved under the biosimilar approval pathway Biologics Price Competition and Innovation Act (BPCIA ) in 2014 [48]. Under this act, protection for the reference epoetin lasts 4 years from a biosimilar agent [49]. Specifically for management of anemia, there are at least six trials registered using biosimilar agents by the two companies aiming to introduce biosimilars in the US [50, 51] in both dialysis and non-dialysis-dependent CKD patients in different stages of completion. The Food and Drug Administration has a stringent and time-consuming process for biosimilar agents and this may slow down introducing more biosimilars into the US market. This cautious approach is supported by an increase in the prevalence of PRCA in countries that have used biosimilars for several years [52]. Though production and use have not been as controlled as the original epoetins even in Europe, reassurance can be had from the European experience of almost a decade of use, especially in a highly regulated and stringent environment. That and with a regimented approach paralleling that of the original biologic agents, there is not a strong argument to keep biosimilars out of reach of US nephrologists’ use in the near future.
For several years now and in several countries, epoetin-like peptides have been developed without licensing and stringent production standards. They differ dramatically in their structure and hence also in their clinical efficacy and side-effect profile [53]. There is a concern for increased incidence of PRCA with these products due to potentially lower production standards and for various other adverse effects due to unregulated or clandestine use, but due to a mismatch between demand and affordability, these are probably the only means of access to ESAs in many countries [54]. More data are awaited on these “copy” epoetins.
Treatment of Anemia with rHuEPO and Its Derivatives: Efficacy and Safety
The efficacy of rHuEPO in correcting anemia in CKD and ESRD has been successfully established shortly after the manufacturing of the molecule for therapeutic utilization. After rHuEPO administration, a measurable increase in reticulocytes is seen in 7–10 days with an increase in hemoglobin following in 2–3 weeks. A concomitant drop in serum iron indices is evident unless iron is replenished simultaneously (more about the role of iron below). The widespread availability of rHuEPO in dialysis patients has resulted in a rapid uptick in its clinical application, and a broadening of its recipient base from dialysis patients to the much larger group of patients with non-dialysis-dependent CKD, and separately to patients with anemia caused by chemotherapy. The initial enthusiasm surrounding rHuEPO led to a belief that complete therapeutic correction of anemia of CKD and normalization of blood hemoglobin concentrations using this agent was possible and beneficial; hence a series of randomized controlled clinical trials were designed to test this hypothesis.
The approval of ESAs was based on small clinical trials and other observational studies showing clinical benefit along with avoidance of repeated transfusions [55]. Encouraged by these data, the first prospective randomized trial to assess the possibility of benefits in normalizing blood hemoglobin concentration was the US Normal Hematocrit (Hct) trial [56]. The goal of the study was to ascertain if a higher target Hct achieved with ESA therapy was indeed better. Dialysis patients were randomized into two groups with different target Hct (30% vs. 42%), and followed until the development of the composite primary end point of death or first nonfatal myocardial infarction. The study was prematurely halted due to a higher proportion of patients in the higher Hct target group reaching the primary end point, thus obviating the possibility of any benefit in “normalizing” the Hct and suggesting that treatment with rHuEPO to a normal Hct target may in fact be detrimental. In addition to the primary end point, other clinical end points such as vascular access thromboses also suggested detrimental effects from the normal Hct treatment paradigm . Another study evaluating the change in the left ventricular volume index in dialysis patients with different Hgb targets (9.5–11.5 g/dL vs. 13.5–14.5 g/dL) detected no difference in outcome between the two groups, suggesting a lack of benefit from normalizing Hgb levels to another hypothetical treatment target [57]. Along with the clinical trials conducted in dialysis populations, several trials were designed to test similar hypotheses in anemic patients with non-dialysis-dependent CKD. The Cardiovascular Risk Reduction by Early Anemia Treatment with Epoetin Beta (CREATE) trial was an international randomized controlled clinical trial of patients with advanced non-dialysis-dependent CKD, which studied the incidence of cardiovascular events in two groups assigned to different Hgb targets and different timings of epoetin-β initiation [58]. No differences in primary end points were detected and, interestingly, the group initiating epoetin-β earlier experienced a shorter time to dialysis. The Correction of Hemoglobin and Outcomes in Renal Insufficiency (CHOIR) trial was another large randomized controlled clinical trial designed to address the effect of different Hgb treatment targets (11 g/dL vs. 13 g/dL) on clinical events [59]. The group assigned to the higher Hgb target experienced a significantly higher rate of the composite primary end point (congestive heart failure, hospitalizations, stroke, or myocardial infarction) compared to the low target group. Also, there was not any improvement in the quality of life as perceived by the participants in the higher Hgb target. Similar results were reported in the Trial to Reduce Cardiovascular Events with Aranesp Therapy (TREAT) trial using darbepoetin, in that the darbepoetin-treated arm experienced a significantly higher incidence of stroke compared to the placebo arm, and without a benefit in the other study end points [60]. Benefits from using ESAs were also examined in trials conducted in non-CKD patients, especially those with heart failure. In the Reduction of Events by Darbepoetin Alfa in Heart Failure (RED-HF) trial , patients with systolic heart failure were administered darbepoetin in an effort to study its effect on a composite primary end point of death or hospitalizations related to heart failure [61]. Interestingly, no differences were seen in the treatment group compared to placebo and a higher incidence of thromboembolic events in the darbepoetin group led to a halt in design of large trials using ESAs in heart failure. This is in contrast to the trials conducted with intravenous iron (IVI) in heart failure (discussed below).
From these clinical trials, it has become clear that normalization of Hgb using treatment with rHuEPO is still fraught with difficulties: it does not result in the expected clinical benefits, it could be detrimental, and last but not least, it is very expensive. Hence, current guidelines suggest maintenance of Hgb in the 10–12 g/dL range with focus on avoiding transfusion, recognizing the benefits of rHuEPO therapy in sparing blood transfusions in patients with severe anemia of CKD, but also acknowledging a lack of high-quality evidence on the ideal Hgb level for these interventions. It also remains unclear if the Hgb treatment targets established in the wake of the rHuEPO trials are valid end points for therapeutic strategies employing agents with distinctly different mechanisms of action (vide infra). Post-hoc analyses have suggested that the adverse effects seen in the high-Hgb target arms of the rHuEPO trials may have been attributable to the higher doses of rHuEPO and/or the relative iron deficiency induced by this strategy [62]; hence the ideal therapeutic targets for other anemia therapies will have to be evaluated in dedicated clinical trials.
ESA Hyporesponsiveness
One of the common problems with ESA therapy in dialysis patients is hyporesponsiveness to ESAs. Hyporesponsiveness is defined as inability to reach the target hemoglobin level irrespective of the ESA dose and continued need for supra-normal doses of ESAs [63, 64]. This is most commonly due to the chronic state of inflammation seen in dialysis patients as part of the malnutrition-inflammation complex syndrome (MICS) [65–67]. MICS has far-reaching consequences beyond ESA hyporesponsiveness [68]. Dialysis patients have several other exclusive causes (tunneled cuffed catheters, impure dialysate, etc.) that make this clinical predicament common [69, 70]. Apart from MICS, several other causes for ESA hyporesponsiveness have been identified (Table 5.2). A consistent finding is a low serum albumin in many patients with hyporesponsiveness; in fact, serum albumin is a strong predictor of ESA response in these patients [71]. Addressing the etiology of hyporesponsiveness (when possible) is usually curative of the condition.
Table 5.2
Common causes of ESA hyporesponsiveness
Cause | Example |
---|---|
Dialysis-related | |
Aluminum toxicity, β2-microglobulin amyloidosis | |
Severe secondary hyperparathyroidism [211] | |
Inadequate dialysis [212] | |
Bone marrow disorders | Myelofibrosis, sickle cell anemia, pure red cell aplasia [213], iron restriction/bleeding |
Malnutrition | Vitamin B12 deficiency, l-carnitine, folic acid, pyridoxine |
Medications | Dapsone, Primaquine, nitrofurantoin, Sirolimus, ACE inhibitors |
Inflammation | MICS [68], SLE, failed kidney transplant |
Chronic infections: Osteomyelitis, cellulitis, HIV |
PRCA
In 2002, a serious side effect, PRCA was reported in patients receiving SQ epoetin-α [19]. This condition is characterized by severe hypo-proliferative anemia caused by the lack of a functional EPO response resulting from an immune response against circulating EPO molecules [72]. Though several causes were proposed (including storage quality lapses and syringe material breakdown) [73], the finding of SQ administration was a stark constant in the reported cases. As mentioned above, PRCA has also been described in conjunction with other ESAs, and remains a dreaded complication of such therapies [19, 74]. At this time, evidence suggests that the development of antibodies against conventional rHuEPO can also neutralize native EPO leading to PRCA, but how this can lead to PRCA in dialysis patients [72], most of whom have minimal native EPO production, remains unanswered. There is evidence that native EPO is not completely lost even in patients with ESRD and can be augmented in acute anemia but not in chronically low Hct states [75, 76]; this “basal” EPO production may even contribute to post-transplant erythrocytosis [77, 78]. Hence, it is possible that this minimal EPO production is essential in avoiding PRCA rather than hemoglobin homeostasis. Treatment of PRCA consists of blood transfusions and immune suppression; a report suggested that an ESA with molecular structure distinctly different from EPO such as peginesatide (vide infra) may be successful in alleviating PRCA [79]. PRCA typically subsides after kidney transplantation, presumably because of the immune suppression received.
Peginesatide
A novel molecule, peginesatide , was developed as an agent that would activate the EPO-R but not have any structural similarity to EPO or its synthetic derivatives [79]. It is a pegylated, dimeric peptide that showed good in vitro results on erythroid progenitor cell lines and with animal studies showing encouraging data, a small group of dialysis patients with previously diagnosed pure red cell aplasia (PRCA) were given peginesatide in an open label trial [80]. This led to three large clinical trials to study the applicability of peginesatide to both CKD and dialysis patients. In the Peginesatide for the Correction of Anemia in Patients with Chronic Renal Failure Not on Dialysis and Not Receiving Treatment with Erythropoiesis-Stimulating Agents) (PEARL 1 and 2) trials, CKD patients were randomized to receive either darbepoetin in the control arm or peginesatide in the treatment arm [81]. Though the efficacy of peginesatide was similar to that of darbepoetin in correcting the Hct, the study was halted due to an unacceptably high incidence of cardiovascular events and death. The Efficacy and Safety of Peginesatide for the Maintenance Treatment of Anemia in Patients with Chronic Renal Failure Who Were Receiving Hemodialysis and Were Previously Treated with Epoetin (EMERALD 1 and 2) trials which was conducted with peginesatide in dialysis patients also reported similar results of efficacy when compared to epoetin [82]. Interestingly, the cardiovascular adverse events and mortality rates in both groups in these trials were similar. This discrepancy in the adverse events with peginesatide in the two sets of trials are yet unexplained. As mentioned above, peginesatide has been sparingly used in patients with anti-erythropoietin antibodies with clinical benefit [80]. That said, at this time, the side-effect profile has relegated peginesatide to an “also-ran” in the race for the next agent for anemia management.
Iron
Iron is a key ingredient in normal erythropoiesis. A typical daily adult diet contains about 15–20 mg of elemental iron ; only 10% of this is absorbed, which is usually sufficient to offset iron losses from epithelial desquamation and sporadic microscopic bleeding. Most of the iron needed for daily erythropoiesis, about 20–30 mg, is reclaimed from senescent RBCs by macrophages. Iron in the diet is absorbed only in the ferrous form (the ferric form has to be converted to the ferrous form and ascorbic acid facilitates this conversion) through the enterocytes via transporters and then into the plasma through ferroportin-1 (FP-1) [83]. Iron is delivered in the ferric form to transferrin [84] that in turn delivers the iron by binding directly to a transferrin receptor (TfR-1) on the surface of the erythroblast which is then internalized into a siderosome [85]. The ferric form is then reduced to the ferrous form and utilized for heme synthesis within the mitochondria and the transferrin is recycled back into the circulation [84–86].
Recently, hepcidin has been identified as a key player in iron metabolism. Hepcidin is produced by the liver in response to various stimuli [85, 87–89] (with inflammation being a key one) and was initially identified as an antimicrobial peptide. Indeed, hepcidin is possibly protective in the setting of a severe infection or bacteremia by restricting the availability of iron for the microorganism’s enzymes that use iron as a co-factor. Hepcidin blocks the absorption of iron from the enteral tract or the release of iron from the reticulo-endothelial cells by binding to FP-1 leading to its internalization and degradation [90]. Hepcidin inactivation leading to juvenile hemochromatosis, a particularly severe form of hereditary hemochromatosis and the role of mutation in the coding gene, HJV, has been well described [91–93].
Iron Replacement in Anemia
Iron replenishment is an essential aspect in the management of anemia of various etiologies; mostly when iron deficiency is the main cause of the anemia, but also in the context of other treatments, such as with rHuEPO. In addition to the “usual” iron losses, CKD patients may also lose iron as a result of more frequent gastrointestinal or other bleeding, frequent blood testing or blood losses related to hemodialysis [94]. Coupled with ineffective iron absorption through the gastrointestinal tract in dialysis patients [95], this loss of iron can amount to a negative iron balance of 1.5–3 g per year [96]. Unless at least a similar amount of iron is supplemented, ESA treatment is expected to be ineffective. Also, with rHuEPO administration and enhanced erythropoiesis, there is significant decrease in iron stores and a lack of replenishment leads to suboptimal and eventually arrested response to rHuEPO or its derivatives. The traditional route of administration for iron has been oral. Ferrous sulfate in oral preparations has been effective in patients with iron deficiency who are devoid of chronic inflammation and liver disease, but less so in patients in whom hepcidin prevents effective restoration of iron stores. In such patients intravenous iron (IVI) preparations have gained popularity (Table 5.3), especially in cases where this route is also convenient and practical, such as chronic HD patients and this is supported by evidence of clinical benefit and cost savings [97–99].
Table 5.3
IVI agents available in the US
Brand name | IVI product | Company | Molecular weight (MW) | Maximum one time dose | Test dose |
---|---|---|---|---|---|
INFeD® | Iron dextran (low molecular weight) | Watson pharmaceuticals | 96 kDa | 1000 mga | Yesc |
Dexferrum® | Iron dextran (high molecular weight) | American regent labs | 265 kDa | 100 mgb | Yesc |
Ferrlecit® | Iron gluconate | Watson pharmaceuticals | 38 kDa | 125 mga | No |
Venofer® | Iron sucrose | American regent labs | 43 kDa | 500 mga,d | No |
100 mgb | |||||
Feraheme® | Ferumoxytol | AMAG pharmaceuticals | 750 kDa | 510 mga | Noc |
Ferinject® | Ferric Carboxymaltose | Vifor pharmaceuticals | 150 kDa | 1000 mga | No |
Assessment of Iron Stores and Therapeutic Targets
The gold standard method for assessment of iron stores continues to be the invasive semi-quantitative bone marrow staining for iron but this is rarely done [100, 101]. A liver biopsy and staining to assess the liver iron stores is also done only in extreme circumstances, although an indirect assessment via the Superconducting Quantum Interference Device (SQUID) may offer an alternative [102]. In clinical practice, iron stores are assessed most often by measuring serum ferritin and transferrin saturation (TSAT) but since inflammation to some degree is present in a large majority of patients, relying on these parameters to initiate IVI is fraught with difficulty as ferritin and TSAT are inversely affected by inflammation [103–105]. Since the lower limits of both ferritin and TSAT have low sensitivities and there is an appreciable response to IVI in patients with normal or even moderately elevated levels of either one [97, 106, 107], their utility in triggering replacement is questionable. Absolute iron deficiency states are consistent with very low ferritin levels, even in the presence of inflammation but this association ceases to regulate ferritin levels once a minimum amount of iron becomes available hence increasing the specificity of a very low ferritin level [108, 109]. Use of ferritin to withhold IVI is again not reliable. Even when IVI replacement guidelines were strictly adhered to, a majority of patients were noted to have iron overload on hepatic imaging [110]. Therefore, dependence on ferritin and TSAT alone is problematic.
Another unique condition seen in patients who are being treated with ESAs is relative iron deficiency. Driven by exogenous ESAs, the bone marrow “strips” the transferrin-bound iron at a rate that exceeds its replenishment. Hence, even though there is not a real body iron deficiency, TSAT can be <20% [111]. In these patients, using the traditional tests is inaccurate at best. Another issue that has been mentioned above is the inhibitory effect of hepcidin on mobilization of iron stores in inflammatory states. This leads to low TSAT but normal or even high ferritin levels. These two situations aptly summarize the handicap associated with using TSAT and ferritin as markers for iron stores [111].
Other indicators such as reticulocyte hemoglobin content (CHr), percentage of hypochromic red cells, or soluble transferrin receptor test (sTfR) have been evaluated as indices for iron stores. CHr is a test that detects the amount of iron available for hemoglobin production. It does not have the bias of other tests that rely on the entire age spectrum of RBCs, and it has shown better accuracy in response to IVI in dialysis patients when compared to ferritin and TSAT [100]. The latter study also established a cutoff level for CHr to be used to guide IVI. Percentage of hypochromic red cells (PHRC) estimates the concentration of Hgb in the RBCs and has comparable specificity for detecting iron deficiency states [107, 112] but is not useful when blood samples have a long transit time to the central laboratories as is the case in most large dialysis companies [111] in the US. Similarly, using sTfR as a diagnostic tool relies on the correlation of increased sTfR on the surface of erythroblasts in iron-deficient states but this is confounded by ESAs; ESAs also can lead to elevated sTfR levels by virtue of an increased cell mass. As a result, the results of using this test are mixed in studies of ESRD patients [113, 114]. The newer tests for iron estimation have generated low interest thus far and, at this time, we are still reliant on TSAT and ferritin to determine the necessity of IVI in the US.
Optimal target iron levels have been deduced after observational studies have shown that optimal erythropoiesis is seen in dialysis patients when TSAT is 30–50% and ferritin is as high as 1200 ng/mL [115]. Also, ferritin levels <2000 ng/mL may not be associated with iron overload as evidenced by imaging studies of the liver [105]. The predicament with this is the association of high ferritin with increased dialysis morbidity, iron overload [116], and mortality [117], though one study suggested that the association of elevated ferritin with poor outcomes is confounded by markers of inflammation and malnutrition [118]. In summary, current K/DOQI guidelines recommend individualization of IVI administration when serum ferritin is >500 ng/mL [105].
Benefits of IVI
There is no doubt about the hematological benefits of IVI in both non-dialysis and dialysis patients. Not only do patients have a robust increment in Hct levels but there is a lower ESA requirement when IVI is concomitantly given [97]. An important question is whether or not such hematologic benefits extend to improved clinical outcomes.
Cardiovascular benefits of correction of iron deficiency have been well studied and documented. The first study performed in patients with congestive heart failure (CHF) showed improved cardiac function and New York Heart Association (NYHA ) classification [119] with SQ EPO. In another study conducted in CHF patients, IVI also improved measures of functional status and quality of life indices along with fewer hospitalizations [120]. Similar results were also found in the ferric carboxymaltose evaluation on performance in patients with iron deficiency in combination with chronic heart failure (CONFIRM-HF) trial [121]. Compared to a placebo, patients on IVI performed significantly better on subjective scores and had fewer hospitalizations as well; differences between the two groups were seen halfway into the study period and continued going forward.
An additional benefit of iron supplementation is a lowering of ESA requirements. Lower ESA requirements were seen in dialysis patients when given IVI compared to oral iron [122]. A follow-up study to the Dialysis Patients’ Response to IVI with Elevated Ferritin (DRIVE) study showed significant reduction in both ESA requirement and cost of anemia management in response to IVI [98, 99]. Whether this translates into better clinical outcomes still needs examination. Cause for skepticism comes from finding poor correlation between higher hemoglobin levels from ESAs’ administration and equivocal clinical outcomes in heart failure [61]. Currently, a large trial is underway to study all-cause mortality in dialysis patients receiving IVI; the results are keenly awaited to shed more light on all-cause mortality and non-fatal cardiovascular events of IVI using iron sucrose [123].
Safety Profile of IVI
A major conundrum with IV iron preparations is the non-physiologic nature of this route, resulting in various complications ranging from allergic reactions and anaphylaxis to potential for oxidative stress, kidney damage, and iron overload [124, 125].
Iron overload has been a major issue ever since transfusions were used to treat anemia of CKD with wide ranging cellular effects [126, 127]. With the emergence of IVI as a pivotal tool to tackle anemia of CKD, concerns for iron overload are legitimate. As mentioned earlier, ferritin levels can help with starting IVI but may not be accurate when used to screen for withholding IVI in patients.
Short-term side effects of IVI administration are usually mild and include nausea, hypotension, and/or diarrhea. The more severe reactions such as urticaria, anaphylactic shock, or laryngeal edema are more common with iron dextran preparations due to the dextran component and there is some evidence that the larger dextran component leads to more frequent reactions [128–131]. This necessitates a test dose administration and careful monitoring. Prospective studies to specifically assess adverse effects have been few so far; retrospective analyses of previously conducted large clinical trials will help to study causality and assess safety profiles of IVI. A large double-blinded study noted that hemodialysis patients, when exposed to sodium ferric gluconate, had similar levels of anaphylactic reactions as the placebo group [132]. This, along with a similar safety profile for iron sucrose [133], suggests that non-dextran-based IVI is relatively safe and does not need a test dose.
In contrast to the immediate and/or short-term side effects, studying long-term adverse effects are obviously more difficult. Sparse data exist that are sufficiently robust to indicate a causality link between IVI and adverse effects, though the Ferinject assessment in patients with Iron Deficiency Anemia and Non-Dialysis-Dependent Chronic Kidney Disease (FIND-CKD) found similar event rates in both the low and high ferritin target groups treated with IV ferric carboxymaltose [134]. There are conflicting data on IVI and association with risk of death and mortality rates [135, 136]. A more recent observational study found a lower risk of death in dialysis patients who were given less than 400 mg IVI when compared to those who were either given more or not given any IVI [118]. IVI has been studied in comparison to oral iron in clinical trials of non-dialysis CKD patients and has been found to be similar in efficacy and in incidence of adverse reactions such as cardiovascular and thromboembolic events [134, 137]; the type of IVI used (ferric carboxymaltose or iron isomaltoside) did not significantly affect adverse events. This is in contrast to a smaller single center clinical trial that showed a higher incidence of cardiovascular events and infections in the IVI group compared to the oral iron group leading to early termination of the trial [138]. To ascertain the true effect on adverse events, much larger trials with IVI are necessary.
An association of interest with IVI is the risk of infection. Iron is a preferred element for several bacteria including Staphylococcus species [139]. Even oral iron has been shown to support bacterial growth in vitro [140], hence this is a big concern especially because of the scale of IVI use in dialysis patients worldwide. A small prospective study did not show any correlation with infections [141], but a much larger observational study showed a small but specific link between iron dosing and infection-related hospitalizations; risk was highest in those individuals who had a high serum ferritin and received a higher dose of IVI [142]. The same study also found an association between infections and bolus dosing. Infections and infection-related hospitalizations were also noted to be higher in patients treated with IVI compared to oral iron in non-dialysis CKD patients in a recent trial [138]. In summary, it is advisable to withhold IVI in patients with systemic/extensive bacterial infections and to have a measured approach in general considering that dialysis patients are vulnerable to infections by nature of their chronic illness.
A long-term potential side effect of IVI is nephrotoxicity due to the cytotoxic effects of iron-induced oxidative stress and mitochondrial injury [143–145], which has been suggested with iron sucrose and ferric gluconate [143]. A head-to-head comparison study noted a higher degree of proteinuria with iron sucrose compared to ferric gluconate [146]. Due to the strong correlation of proteinuria with CKD progression to ESRD and to cardiovascular disease, there is a legitimate concern for long-term IVI safety. When studied prospectively if IVI forms did indeed affect proteinuria upon repeated exposure, iron sucrose was found to have a sustained proteinuric effect in CKD patients when compared to ferric gluconate [147]. Taken together, these findings suggest that IVI has to be used judiciously in non-dialysis-dependent CKD patients.
A new development in the US that might be detrimental to ESA use and increase IVI use has been the introduction of a “bundled” payment for IV iron and ESAs in 2011 by Center for Medicare and Medicaid Services (CMS) . Following the introduction of this payment system, the mean Hgb and ESA doses declined whereas a higher rate of transfusions and use of IV iron was noted [148]. Whether the potential for a financially motivated increase in IVI administration in the US is indeed leading to changes in practice trends raises further concerns about safety and requires urgent investigation in dedicated clinical trials [70].
Hypoxia-Inducible Factor (HIF Modulation)
HIFs are proteins that play a pivotal role in signal transduction of EPO production (Fig. 5.3). The HIF complex consists of either HIF-1α or an oxygen-sensitive HIF-2α subunit that is associated with a constitutively expressed HIF-1β subunit. Human renal fibroblasts, under hypoxic conditions, activate only HIF-2α and not HIF-1α [149]; this finding has been supported by evidence of activating mutations in HIF-2α leading to erythrocytosis [150]. HIF-2α, in the absence of hypoxia, is hydroxylated at 2 proline residues by proline hydroxylases [151] (2-oxoglutarate superfamily-dependent dioxygenases [152, 153]); this step marks the HIF-2α for binding by von Hippel Lindau (VHL) protein and subsequently for ubiquitination and proteasomal degradation [154]. Proline hydroxylase 2 (PHD2) has been identified as the key enzyme that regulates HIF-2α stability; PHD2 has also been identified in mutations leading to erythrocytosis [155–157]. HIF-2α, in hypoxic states, is not hydroxylated due to inhibition of proline hydroxylase and hence forms a heterodimer with HIF-1β that translocates to the nucleus and binds to the hormone response element (HRE) of EPO and induces EPO transcription [158]. Ergo, PHD2 inhibitors represent a novel target for therapeutic intervention and this opportunity has been pursued to develop therapeutic agents that can stabilize the HIF complex and hence positively influence EPO production.


Fig. 5.3
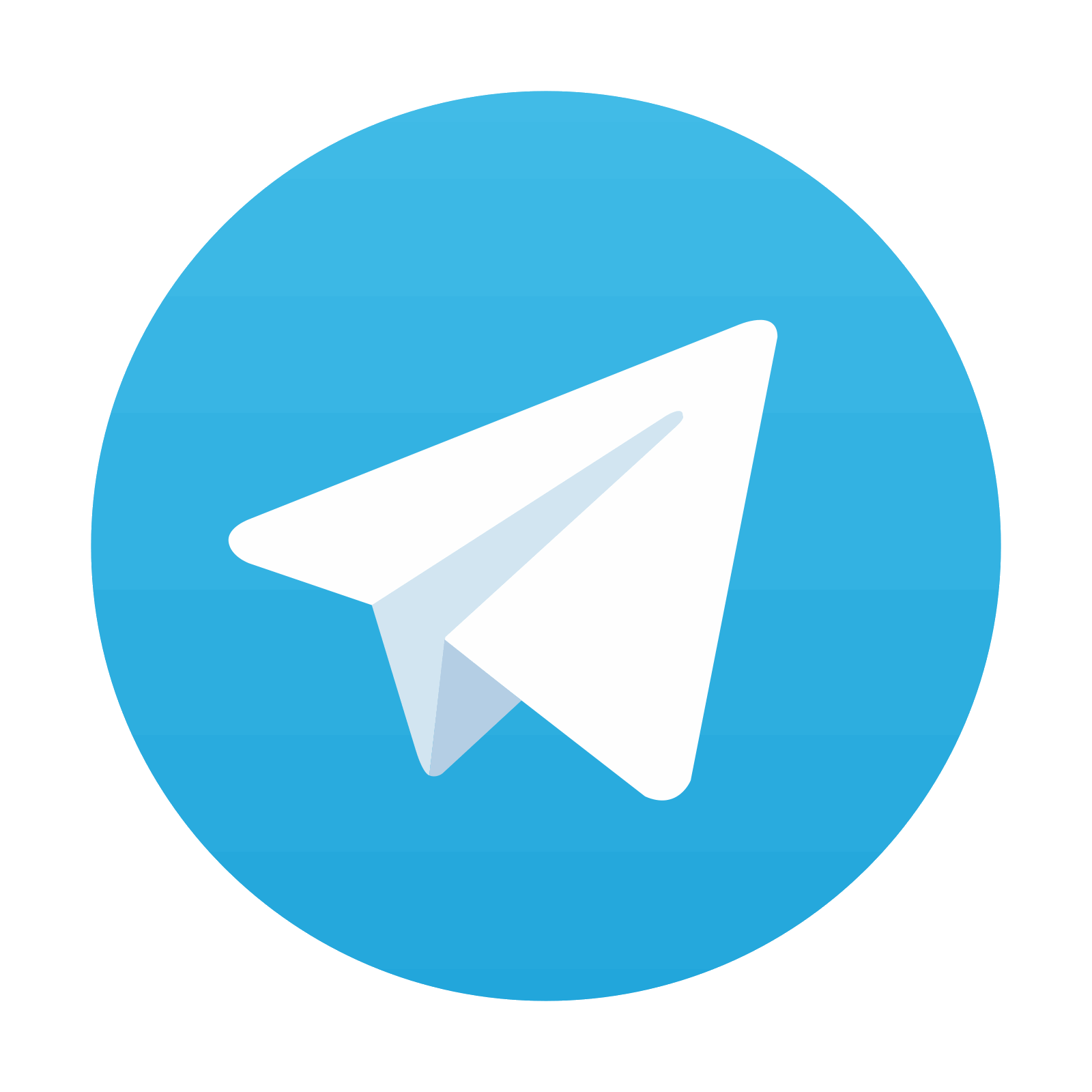
EPO production signaling pathway. Under normoxic conditions, HIF-2α is hydroxylated at 2 proline residues by PHD2 and this marks HIF-2α for VHL protein binding. The VHL protein and its associated ubiquitin ligase attach ubiquitin to HIF-2α and hence relegate it for proteasomal degradation. Under hypoxic conditions, HIF-2α dimerizes with HIF-1β, a constitutively expressed protein, and the heterodimer translocates to the nucleus and binds to the hypoxia response element (HRE) of EPO to increase EPO gene transcription
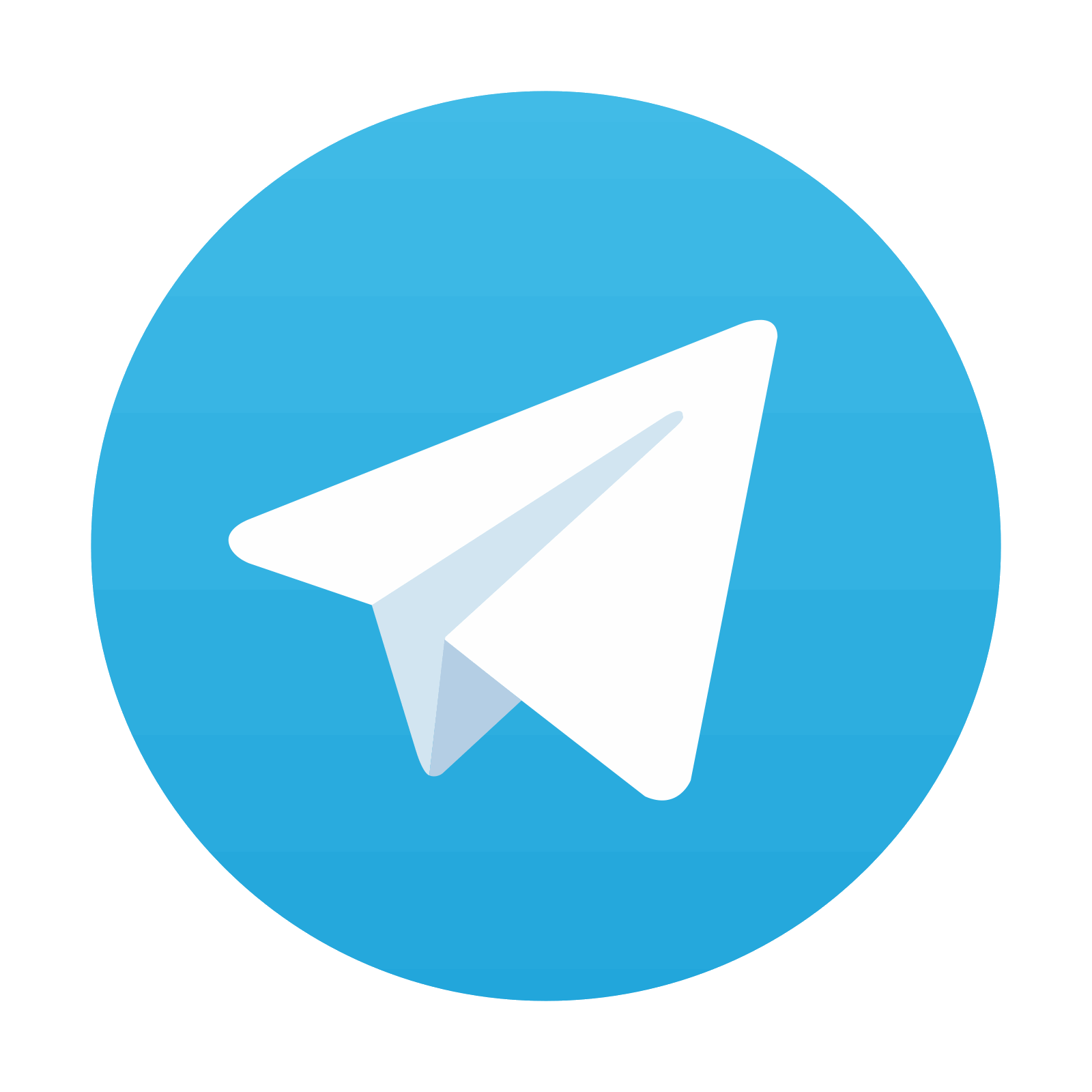
Stay updated, free articles. Join our Telegram channel
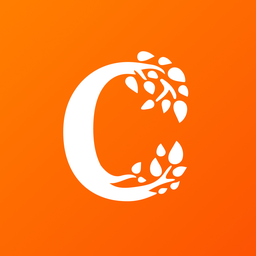
Full access? Get Clinical Tree
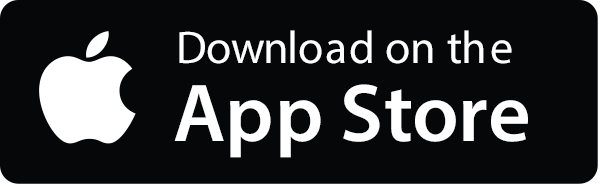
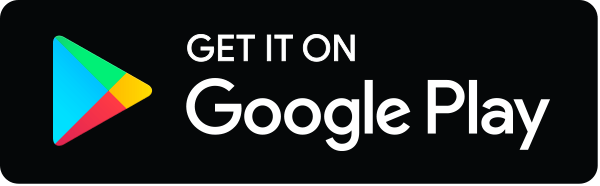
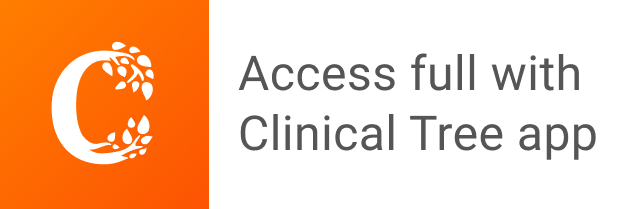