Abstract
Peroxisomes are ubiquitous subcellular organelles that are found in essentially all animal and plant cells with the exception of mature anucleated erythrocytes. They carry out many essential biochemical processes, both catabolic and anabolic. Thus, deficiency of numerous peroxisomal proteins essential for structural integrity and metabolic functions causes human disease. These disorders are grouped as either peroxisome biogenesis disorders or isolated peroxisomal protein/enzyme deficiencies. With increased utilization of DNA sequencing as a diagnostic tool, the clinical spectrum of these disorders has expanded, and additional disease genes have been reported. Ultrastructural analysis of hepatic tissue led to the initial association of peroxisomes with human disease. However, not all peroxisomal diseases have liver involvement and thus would not be expected to present to the hepatologist. In this chapter, the focus will be on those diseases in which there is a hepatic component (see Table 37.1).
Introduction
Peroxisomes are ubiquitous subcellular organelles that are found in essentially all animal and plant cells with the exception of mature anucleated erythrocytes. They carry out many essential biochemical processes, both catabolic and anabolic. Thus, deficiency of numerous peroxisomal proteins essential for structural integrity and metabolic functions causes human disease. These disorders are grouped as either peroxisome biogenesis disorders or isolated peroxisomal protein/enzyme deficiencies. With increased utilization of DNA sequencing as a diagnostic tool, the clinical spectrum of these disorders has expanded, and additional disease genes have been reported. Ultrastructural analysis of hepatic tissue led to the initial association of peroxisomes with human disease. However, not all peroxisomal diseases have liver involvement and thus would not be expected to present to the hepatologist. In this chapter, the focus will be on those diseases in which there is a hepatic component (see Table 37.1).
Liver Disease | Absence of Liver Disease |
---|---|
Peroxisome biogenesis disorders – Zellweger spectrum (PEX1, PEX2, PEX3, PEX5, PEX6, PEX10, PEX11 beta, PEX12, PEX13, PEX14, PEX16, PEX19, PEX26) | Peroxisome biogenesis disorders – Rhizomelic chondrodysplasia punctata (RCDP) type 1 (PEX7) |
Isolated peroxisomal protein/enzyme deficiencies | Isolated peroxisomal protein/enzyme deficiencies |
Acyl-CoA Oxidase 1 (ACOX1) | RCDP Type 2 (GNPAT) |
Acyl-CoA Oxidase 2 (ACOX2) | RCDP Type 3 (AGPS) |
PMP70 (ABCD3) | RCDP Type 4 (FAR1) |
D-Bifunctional Protein (HSD17B4) | RCDP Type 5 (PEX5 domain encoding the protein region interacting with PEX7) |
αMethylacyl-CoA Racemase (AMACR) | X-linked adrenoleukodystrophy (ABCD1) |
Bile acid-CoA:amino acid N-acyltransferase deficiency (BAAT) | Adult Refsum disease (PHyH) |
Acyl-CoA binding domain 5 deficiency (ABCD5) |
In the disorders listed in column 1, liver disease is secondary to hepatic peroxisomal abnormalities. Two additional peroxisomal disorders may come to the attention of the hepatologist for more complex reasons. In primary hyperoxaluria type 1 (PH1), oxalate produced by the liver causes renal disease but not liver disease; however, treatment may require a combined liver/kidney transplant. In CADDS (contiguous ABCD1 DXS1357E deletion syndrome), the combined lack of peroxisomal ABCD1 and the endoplasmic reticulum protein BCAP31 produces a phenotype that presents with liver dysfunction and cholestasis.
In the disorders listed in the first column of Table 37.1, liver disease is secondary to hepatic peroxisomal abnormalities. Two additional peroxisomal disorders may come to the attention of the hepatologist for more complex reasons. In primary hyperoxaluria type 1 (PH1), oxalate produced by the liver causes renal disease but not liver disease; however, treatment may require a combined liver/kidney transplant. In CADDS (contiguous ABCD1 DXS1357E deletion syndrome), the combined lack of peroxisomal ABCD1 and the endoplasmic reticulum protein BCAP31 produces a phenotype that presents with liver dysfunction and cholestasis.
Peroxisome Structure and Functions
General Aspects of Peroxisomes
Peroxisomes were first identified in renal proximal tubule cells by J. Rhodin, a Swedish graduate student, in 1954 [1]. Initially called microbodies, these organelles were studied intensively by de Duve and co-workers. Because they contained enzymes that both produced (e.g., amino acid and urate oxidases) and degraded (e.g., catalase) hydrogen peroxide, de Duve proposed the name peroxisomes [2]. Peroxisomes range in size from about 0.1 μm (microperoxisomes of intestine and brain) up to 1.0 μm (characteristic of hepatic and renal peroxisomes; range: 0.2–1.0 μm).
The peroxisomal membrane is a single lipid bilayer. The organelle’s matrix is finely granular, but microcrystalline cores of urate oxidase are present in hepatic peroxisomes of rodents. Humans lack urate oxidase and thus cores are not found in human peroxisomes. Unlike chloroplasts and mitochondria, peroxisomes contain no DNA. An early hypothesis speculated that all three organelles evolved from endosymbionts. However, more recent analysis of the peroxisome proteome suggests an endogenous origin from within the endomembrane system [3]. Since the discovery of peroxisomes, numerous membrane proteins and matrix enzymes have been identified. Much research on peroxisomes has been fueled by the identification of patients whose cells lack either normal appearing organelles or one or more peroxisomal metabolic functions.
Biogenesis of Peroxisomes
The processes involved in de novo formation and division of peroxisomes have been intensively studied and debated. Studies done primarily with yeast mutants and cells obtained from human patients with peroxisomal biogenesis disorders have substantially increased our understanding of the proteins and processes involved. Proteins required for peroxisome biogenesis are called peroxins or PEX proteins, and are encoded by PEX genes. See Ma et al. [4] and Argyriou et al. [5] for an overview of peroxisome assembly in humans.
Peroxisomes can form via a de novo pathway, or by fission of pre-existing peroxisomes. The current model of de novo peroxisome biogenesis in mammalian cells begins with the formation of pre-peroxisomes. Vesicles derived from both endoplasmic reticulum and mitochondria are now thought to be involved in this process [6]. The peroxisomal membrane protein PEX16 is co-translationally targeted to endoplasmic reticulum membranes. Other membrane proteins such as PEX3 and PEX14 target to mitochondria. Fusion of these two organelle-derived vesicles yields pre-peroxisomes. By importing membrane proteins and matrix enzymes, pre-peroxisomes mature into metabolically functional peroxisomes. Mature peroxisomes can then divide by fission, using PEX11 proteins (PEX11 α, β, γ), yielding daughter peroxisomes that continue to grow by importing membrane and matrix proteins. It is likely that the two processes of new membrane formation and fission occur simultaneously to generate new peroxisomes in cells. PEX11β is widely expressed, is essential for peroxisome fission, and its molecular mechanisms are reasonably well characterized [7]; the functions of PEX11α and γ are less well understood. The latter are tissue specific and mainly expressed in liver.
Peroxisomal matrix enzymes are imported into the growing organelle by the coordinated action of multiple peroxins (Figure 37.1). Two peroxins, PEX5 and PEX7, are cytoplasmic receptors for proteins destined for entry into peroxisomes. Alternative splicing yields two isoforms of PEX5 called “long” and “short” forms. Most matrix proteins are targeted by a tripeptide sequence, referred to as peroxisome targeting signal 1 (PTS1), which binds to both forms of PEX5. The originally described PTS1 sequence, located at the carboxy terminus of a protein, consisted of serine-lysine-leucine, but several conserved variants have subsequently been identified. A smaller number of peroxisomal enzymes enter the organelle via PTS2, a nine amino acid sequence near, but not at, the amino-terminus. Of the seven human proteins with a putative PTS2, five conform to the consensus RΦXXΦXX(H/Q)Φ, where “X” is any amino acid and “Φ” designates a hydrophobic residue [8]. PEX7 is the cytoplasmic receptor for PTS2-containing proteins, and the long form of PEX5 is a required co-receptor for this process. Some matrix proteins contain neither PTS1 nor PTS2, and it has been suggested that they enter the organelle either via an internal PTS, or by a “piggy-back” mechanism in which they bind to a PTS1-containing protein and concomitantly enter the peroxisome. Cytoplasmic PEX19 acts as a receptor, as well as a chaperone, for peroxisomal membrane proteins. Peroxisome targeting signals for membrane proteins are less well defined than those for matrix proteins. No consensus sequences have been identified. Rather, the signal seems to involve a group of basic amino acids in an α-helical configuration adjacent to a hydrophobic domain [9].
Figure 37.1 Peroxisome Biogenesis. A simplified diagram of PEX protein function in peroxisome protein import (right) and import of peroxisome membrane proteins (PMP) (left). Proteins destined for the matrix carry either a peroxisome targeting signal 1 (PTS1) or 2 (PTS2). The receptor for PTS1 proteins is PEX5, either the long (PEX5L) or short (PEX5S) isoform. The receptor for PTS2 proteins is PEX7. PEX7 with its cargo protein binds to PEX5L. Receptors dock at the peroxisome membrane via PEX13 and PEX14 and unload their cargo into the matrix. PEX2, PEX10, and PEX12 assist in recycling of PEX5 and PEX7 back to the cytoplasm via a complex consisting of PEX26, PEX1, and PEX6. De novo formation of peroxisome membranes (not shown) involves budding from the ER of PEX16 and PEX3, and PEX14 and PEX3 from the mitochondria. Left, PEX19 is a cytoplasmic receptor for PMPs during peroxisome growth. PMP-laden PEX19 docks with PEX3 and inserts the cargo into the membrane with the assistance of PEX16. PEX19 recycles back to the cytoplasm. PEX11 (not shown) is a PMP involved in division of existing peroxisomes.
PEX5-PEX7 receptor-cargo complexes dock with an import complex on the peroxisomal membrane consisting of PEX13 and PEX14. After releasing their cargo to the peroxisome matrix, PEX5 is ubiquitinated by ubiquitin ligases PEX2, PEX10, and PEX12. Mono-ubiquitinated PEX5 is subsequently recycled to the cytoplasm, presumably with PEX7, via an export complex containing PEX1, PEX6, and PEX26. Removal of PEX5/PEX7 complexes from the peroxisome membrane is an ATP driven process. PEX1 and PEX6 are members of the AAA (ATPases Associated with various cellular Activities) protein family containing ATP binding and hydrolysis domains and are anchored to the peroxisome membrane through PEX26. PEX1 and PEX6 from a hexameric ring that changes conformation during the ATPase cycle, providing the motion and force needed to extract the receptors [10].
Peroxisome numbers are also regulated by organelle turnover. Pexophagy, or peroxisome turnover, occurs by general mechanisms related to autophagy of cellular components, as well as mechanisms selective for peroxisomes. Deficiencies in various PEX proteins can increase pexophagy, including PEX3, PEX14, PEX1, PEX6, PEX26 [11].
Patients whose cells lack morphologically normal peroxisomal structures have a peroxisome biogenesis disorder-Zellweger spectrum disorder (PBD-ZSD) (reviewed in Argyriou et al. [5]). Skin fibroblasts from severely affected patients may completely lack peroxisomes. More commonly, however, they have vesicular structures containing some peroxisomal membrane proteins. These cells either lack all matrix enzymes (peroxisomal “ghosts”) or exhibit reduced matrix protein import. Autosomal recessive mutations in any one of 13 PEX genes cause PBD-ZSD, whereas mutations in PEX7 cause Rhizomelic Chondrodysplasia Punctata type 1 (see Table 37.1).
Peroxisomal Metabolic Pathways
Peroxisomes carry out vital catabolic and anabolic processes. Many of these pathways involve lipid metabolism, but the spectrum of peroxisomal metabolic function is diverse, including amino acid, purine and polyamine metabolism, as well as maintenance of the cellular redox state. Degradation of very long-chain fatty acids (VLCFA) and methyl branched-chain fatty acids, synthesis of ether-linked phospholipids, primary bile acids and docosahexaenoic acid are among the different pathways of peroxisomal lipid metabolism. Peroxisomal metabolic pathways are depicted in Figure 37.2, and the association between metabolites and disease is shown in Table 37.2.
Figure 37.2 Overview of peroxisome metabolic pathways. Peroxisomal pathways for β-oxidation of straight-chain and branched-chain fatty acids, α-oxidation of phytanic acid, bile acid synthesis, ether phospholipid (plasmalogen) synthesis, pipecolic acid metabolism, glyoxylate detoxification and hydrogen peroxide detoxification are outlined. Long-chain fatty acids (LCFA) and very long-chain fatty acids (VLCFA) are activated to their CoA derivatives extraperoxisomally and then transported into the organelle via ABCD1 (and ABCD2 in certain tissues); Acyl-CoA binding domain 5 (ACBD5) participates in this process. Enzymes necessary for β-oxidation of LCFA-CoA and VLCFA-CoA include acyl-CoA oxidase (ACOX1), D-bifunctional protein (HSD17B4), and 3-ketoacyl-CoA thiolase or the thiolase domain of sterol carrier protein X (ACAA1 or SCP2, respectively). The CoA derivatives of branched-chain fatty acids (BrCFA), phytanic acid, and bile acyl-CoAs are transported into the peroxisome matrix by ABCD3. β-Oxidation of BrCFA-CoA requires branched-chain acyl-CoA oxidase (ACOX2), HSD17B4, and SCP2. These enzymes also convert the CoA thioesters of the bile acid precursors tri- and dihydroxycholestanoic acids (THCA-CoA and DHCA-CoA) into the CoA derivatives of cholic acid (CA-CoA) and chenodeoxycholic acid (CDCA-CoA), respectively. The CoA derivative of phytanic acid (Phy-CoA) is converted to pristanic acid by the α-oxidation pathway, which includes the enzymes phytanoyl-CoA α-hydroxylase (PHYH), hydroxyphytanoyl-CoA lyase (HACL1) and aldehyde dehydrogenase (ALDH3H2). Pristanic acid is further metabolized by the branched-chain β-oxidation pathway; both ACOX2 and ACOX3 can catalyze the first enzymatic step of pristanoyl-CoA degradation. Synthesis of ether phospholipids, including plasmalogens, requires two peroxisomal matrix enzymes: dihydroxyacetone (DHAP) acyltransferase (GNPAT) and alkyl-DHAP synthase (AGPS). The former catalyzes the acylation of DHAP and the latter the displacement of the fatty acid by a fatty alcohol (FAl). Fatty acyl-CoA reductases (FAR1, FAR2) on the peroxisomal membrane are involved in FAl production. Early enzymes in the cholesterol biosynthetic pathway between acetyl-CoA and mevalonate are likely in peroxisomes as well as cytoplasm/endoplasmic reticulum, including thiolase (ACAA1), HMG-CoA synthase (HMGCS1), and HMG-CoA reductase (HMGCR). In contrast, mevalonate kinase (MVK), phosphomevalonate kinase (PMVK), and diphosphomevalonate decarboxylase (MVD), are exclusively cytosolic. The peroxisomal enzyme L-pipecolic acid oxidase (PIPOX) degrades L-pipecolic acid, an intermediate in lysine catabolism. Glyoxylate produced by peroxisomal oxidases is detoxified by the alanine:glyoxylate aminotransferase (AGXT) reaction. Hydrogen peroxide generated by the numerous peroxisomal oxidases is detoxified by either the catalatic (C) or peroxidatic (P) activity of catalase (CAT). Not shown are enzymes for the activation of fatty acids (acyl-CoA synthetases) and α-methylacyl-CoA racemase (AMACR). Also not shown is L-bifunctional enzyme (EHHADH) which catalyzes the second and third reactions in the β-oxidation of dicarboxylic FA-CoAs.
Disorder/Protein Deficiency | ZSD1 | ACOX1 | ACOX2 | DBP1 | AMACR1 | ABCD3 | BAAT6 | ARD | XALD | RCDP | ACBD5 |
---|---|---|---|---|---|---|---|---|---|---|---|
Liver disease | + | + | + | + | + | + | + | − | − | − | − |
Blood | |||||||||||
VLCFA (C26:0, C26:0/C22:0 ratio) | ↑ | ↑ | N | ↑ | N | ↑C26/C22 | N | ↑ | N | ↑ | |
Phytanic acid | N-↑ | N | N | ↑ | ↑ | N | ↑ | N | ↑(RCDP1) | ? | |
Pristanic acid | N-↑ | N | N | ↑ | ↑2 | N | N | N | N | ? | |
C27 bile acid intermediates (DHCA, THCA) | ↑ | N | ↑ | ↑ | ↑3 | ↑ | N | N | N | N | ? |
C24 primary bile acids | ↓ | N | ↓ | ↓ | ↓ | ↓ | Unconj-ugated | N | N | N | ? |
Pipecolic acid | ↑ | N | ↑4 | N-↑ | N | N | N-↑ | N | N | ? | |
Docosohexanoic acid (DHA) | N-↓ | N | ? | ↓ | N | ? | N | N | ? | ? | |
Newborn screening – dried blood spot | |||||||||||
C26:0 lyso-PC levels | ↑ | ↑ | N | ↑ | N | ? | N | ↑ | N | ↑ | |
Urine | |||||||||||
Pipecolate | ↑ | N | ? | N | N | N | N | N | N | ? | |
Oxalate/creatinine | N-↑ | N | ? | N | N | N | N | N | N | ? | |
Erythrocytes | |||||||||||
Plasmalogens | ↓ | N | N | N | N | N | N | N | ↓ | ↓ | |
Fibroblast culture | |||||||||||
VLCFA β-oxidation | ↓ | ↓ | ? | ↓ | N | N | N | ↓ | N | ↓ | |
Pristanic β-oxidation | ↓ | N | N | ↓ | N | ↓ | N | N | N | N | |
Phytanic α-oxidation | ↓ | N | N | ↓ | ↓ | N | ↓ | N | ↓(RCDP1) | N | |
Plasmalogen synthesis | ↓ | N | ? | N | ↓ | N | N | N | ↓ | ? | |
Catalase distribution | C | P | ? | P | P | P | P | P | P | ? | |
Research tools in fibroblast cultures | |||||||||||
Peroxisome import functions | ↓ | N | N | N | N | N | N | N | ↓ | N | |
Peroxisome numbers and morphology | ↓5 | ↓;large | N | ↓;large | N | N | N | N | N | N |
? no data is available
1 Peroxisome functions can be less abnormal or normal in mild and atypical cases.
2 Only the 2 R-stereoisomer of pristanic acid accumulates.
3 Only the 25 R-stereoisomer of DHCA and THCA accumulates.
4 Mild elevation of pipecolic acid in one reported infant (Ferdinandusse et al., 2018).
5 Peroxisomes may be absent in ZSD or present as matrix protein-deficient “ghosts.”
6 Other peroxisome functions were not evaluated in BAAT deficiency but would be expected to be normal.
Abbreviations: ZSD, Zellweger spectrum disorder; ACOX1, acyl-CoA oxidase 1 deficiency; BAAT, bile acid CoA:amino acid N‐acyl transferase deficiency; DBP, D-bifunctional enzyme deficiency; RCDP, Rhizomelic chondrodysplasia punctata; VLCFA, very long-chain fatty acids; N=normal; C=cytoplasmic catalase; P=peroxisomal catalase.
Peroxisomal β-Oxidation
Degradation of the predominant dietary and stored 16- to 18-carbon fatty acids for energy production in humans and other higher organisms takes place in mitochondria. In lower organisms such as yeast, oxidation of all fatty acid species is confined to peroxisomes. The recognition that mammalian peroxisomes are the site for β-oxidation of very long chain fatty acids (>22 carbons) was reported in 1976 [12]. Shortly thereafter, three peroxisomal enzymes catalyzing the four reactions required to chain-shorten fatty acids were found in rat liver. These events marked the beginning of more than four decades of research into the metabolic functions of peroxisomes.
The process by which fatty acids enter peroxisomes is now better understood. Unlike mitochondria, peroxisomes do not use a carnitine acyltransferase mechanism for entry of fatty acids into the organelle. Peroxisomal membranes contain three members (ABCD1-3) of the ATP-binding cassette family of transmembrane proteins thought to be involved in membrane transport [13]. Coenzyme A (CoA) thioesters of saturated VLCFA are thought to be transported into peroxisomes via ABCD1. The peroxisomal membrane protein acyl-CoA binding domain protein 5 (ACBD5) has also been implicated in this process [14]. Activation of a VLCFA to its CoA derivative would thus occur extra-peroxisomally. ABCD2 has been shown to compensate partially for ABCD1 deficiency in experimental models, suggesting that it likely transports structurally similar acyl-CoA substrates [15]. Once inside peroxisomes, saturated, unbranched fatty acyl-CoAs are degraded by the sequential action of three peroxisomal enzymes: acyl-CoA oxidase (ACOX1), D-bifunctional protein (DBP; also known as multifunctional enzyme 2 or MFE2; HSD17B4), and 3-oxoacyl-CoA thiolase (ACAA1), yielding acetyl-CoA and an acyl-CoA shortened by two carbons (Figure 37.2). L-Bifunctional protein (EHHADH; LBP) and SCPX thiolase (SCP2) can partially substitute for MFE2 and thiolase, respectively [16]. Dicarboxylic fatty acids, leukotrienes and other prostanoids, and certain polyunsaturated fatty acids are also partially degraded by this peroxisomal pathway.
While the enzymatic reactions are similar, there are distinct differences between the mitochondrial and peroxisomal pathways of fatty acid β-oxidation. The first mitochondrial reaction is catalyzed by an FAD-containing dehydrogenase that donates its electrons to the respiratory chain, coupling to energy production and ketogenesis in mitochondria. In peroxisomes, the FAD enzyme, ACOX1, is coupled to the production of H2O2 from molecular oxygen. Peroxisomal DBP and LBP contain both enoyl-CoA hydratase and hydroxyacyl-CoA dehydrogenase activities. In mitochondria, these reactions are catalyzed by separate enzymes. Enoyl-CoA isomerase activity has also been attributed to LBP. Distinct mitochondrial and peroxisomal enzymes catalyze the thiolytic cleavage of 3-ketoacyl-CoAs. Studies with knockout mice have suggested that LBP is important in the degradation of dicarboxylic fatty acids [17]. In addition to VLCFA, ACOX1 also oxidizes dicarboxylic and polyunsaturated fatty acids. In PBD-ZSD patients increased dicarboxylic acids in urine, including epoxydicarboxylic acids, have been observed by gas chromatography/mass spectrometry (GC/MS) [18].
Pristanic acid, the 2-methyl-branched-chain fatty acid that is the product of peroxisomal α-oxidation of phytanic acid, and bile acid precursors are catabolized by a pathway consisting of branched-chain acyl-CoA oxidase (ACOX2) and/or pristanoyl-CoA oxidase (ACOX3), DBP, and SCPX thiolase (Figure 37.2). SCPX is a 58 kDa protein (encoded by the SCP2 gene) whose carboxy-terminal 14 kDa is identical to sterol carrier protein 2 (SCP2) and whose amino-terminal 44 kDa has thiolase activity. SCP2, originally thought to be involved in cholesterol movement in cells, may function in peroxisomes as an acyl-CoA binding protein. Interestingly, the carboxy terminal portion of DBP has a high degree of homology to SCP2.
Additional peroxisomal enzymes required for fatty acid β-oxidation include acyl-CoA synthetases for activating long-chain fatty acids and VLCFA, carnitine acetyltransferase (CRAT), carnitine octanoyltransferase (CROT), α-methylacyl-CoA racemase (AMACR; see below), 2,4-dienoyl-CoA reductase (DECR2), trans-2-enoyl-CoA reductase (PECR), and Δ3,5, Δ2,4-dienoyl-CoA isomerase (ECH1). After peroxisomal degradation to carbon chain lengths around C16, these shorter fatty acids are transferred to mitochondria for additional rounds of β-oxidation [16].
Human diseases result from impaired peroxisomal fatty acid β-oxidation. Patients with disorders of peroxisome biogenesis have defective β-oxidation of both VLCFA and branched-chain fatty acids [19, 20]. In these disorders, the absence of normal peroxisomes results in mistargeting and/or cytoplasmic degradation of β-oxidation enzymes. All other peroxisomal metabolic processes are affected as well. Impaired β-oxidation of VLCFA is also found in patients with single enzyme defects of either ACOX1 or DBP, and in X-linked adrenoleukodystrophy (ABCD1 mutation) and ACBD5 deficient patients. Branched-chain fatty acid metabolism and bile acid synthesis are normal in ACOX1 deficiency. Bile acid synthesis, but not pristanic acid oxidation, is reduced in ACOX2 deficiency. DBP-deficient patients have decreased ability to degrade 2-methyl-branched-chain fatty acids and impaired bile acid synthesis. One case of SCP2 deficiency, diagnosed in an adult, was reported in 2006 [21]. Documented single enzyme deficiencies of LBP, ACAA1, and ACOX3 have not yet been reported.
Since peroxisomes lack an electron transport coupled to ATP synthesis, fatty acid β-oxidation in this organelle must serve another purpose. In some cases, the catabolic β-oxidation pathways participate in biosynthetic processes. This is clearly the case for bile acid synthesis from cholesterol. In addition, a requirement for peroxisomal β-oxidation in the biosynthesis of docosahexaenoic acid (DHA; C22:6ω3) was demonstrated [22]. The precursor of DHA, eicosapentaenoic acid (EPA; C20:5ω3), undergoes two cycles of fatty acid elongation, forming C24:5ω3, followed by desaturation to yield C24:6ω3. The latter compound is then chain-shortened by one cycle of peroxisomal β-oxidation, yielding DHA. In other cases, it appears that peroxisomal α-oxidation (below) and β-oxidation functions comprise degradation of compounds that are toxic when present in excess. VLCFA, phytanic acid and many xenobiotics fall into this category.
Peroxisomal α-Oxidation
Intact 3-methyl-branched fatty acids cannot be degraded by β-oxidation. Phytanic acid (3,7,11,15-tetramethylhexadecanoic acid), derived from the phytol side chain of chlorophyll, is the most significant 3-methyl-branched fatty acid in the human diet. Phytanic acid is not synthesized by humans but rather is ingested from ruminant meats and fats (e.g., dairy products) in the diet. Identification of this fatty acid as the compound that accumulated in plasma and tissues of patients with adult Refsum disease (ARD) [23] led to the discovery of the α-oxidation pathway. Patients with ARD develop an adult-onset neuropathy and retinitis pigmentosa without evidence of liver disease.
Entry of the CoA thioesters of 3-methyl-branched-chain fatty acids into peroxisomes requires the transporter ABCD3, also known as PMP70 (peroxisomal membrane protein of 70 kDa) [24] (Figure 37.2). Inside the peroxisomal matrix, phytanoyl-CoA is hydroxylated in an oxygen-requiring dioxygenase reaction catalyzed by phytanoyl-CoA α-hydroxylase (PHYH). Hydroxyphytanoyl-CoA lyase (HACL1) then catalyzes the cleavage of α-hydroxyphytanoyl-CoA to pristanal, a branched-chain fatty aldehyde, and the one-carbon metabolite formyl-CoA. The latter compound rapidly degrades at neutral pH and ultimately yields CO2. Pristanal is then oxidized to pristanic acid (2,6,10,14-tetramethylpentadecanoic acid) via an aldehyde dehydrogenase (ALDH3H2). Pristanic acid must then be converted to its CoA derivative prior to subsequent metabolism, and it has been suggested that this reaction is catalyzed by intraperoxisomal very long-chain acyl-CoA synthetase 1 (ACSVL1; SLC27A2) [25]. The net result of α-oxidation is the decarboxylation of phytanic acid with shift of the position of the methyl group by one carbon, allowing further degradation of pristanoyl-CoA by the branched-chain peroxisomal β-oxidation pathway.
PHYH, like ACAA1, is a PTS2-containing protein, whereas HACL1 contains a PTS1. In disorders of peroxisome biogenesis, neither class of matrix enzymes are targeted properly and defective phytanic acid oxidation and elevated plasma levels of phytanic acid are observed. However, because phytanic acid is solely of dietary origin, plasma levels and total body burden in a given patient reflect both the patient’s age and diet. In some children with defective peroxisome biogenesis, abnormal phytanic acid metabolism was the initial observation, prompting the former term, “infantile Refsum disease.” As discussed below, these patients have multiple peroxisomal metabolic defects and are now considered to be at the milder end of the Zellweger spectrum.
Bile Acid Synthesis
The peroxisome plays a crucial step in the synthesis of mature bile acids. Enzymatic pathways in the endoplasmic reticulum convert cholesterol into trihydroxycholestanoic acid (THCA) and dihydroxycholestanoic acid (DHCA) (C27 bile acid intermediates). Activation of THCA and DHCA to their CoA derivatives is thought to occur mainly in the endoplasmic reticulum [25], and entry of these activated bile acid precursors into peroxisomes proceeds via ABCD3. The side chains of THCA and DHCA resemble 2-methyl-branched-chain fatty acids and undergo a single cycle of β-oxidation in peroxisomes to produce the primary bile acids cholic acid (CA) and chenodeoxycholic acid (CDCA), respectively (Figure 37.2). Both THCA-CoA and DHCA-CoA must first be converted to the correct stereoisomers via peroxisomal methylacyl-CoA racemase (AMACR; see below) before being processed by ACOX2, DBP, and SCPX. The products of β-oxidation are the CoA derivatives of the primary bile acids. Peroxisomal bile acid-coenzyme A:amino acid N-acyltransferase (BAAT) then catalyzes the formation of glycine or taurine conjugates of CA and CDCA which exit the peroxisome by a specific transporter. Elevated C27 bile acid intermediates and decreased C24 primary bile acids are detected in plasma from patients with PBD-ZSD, and in patients with AMACR, ACOX2, DBP and SCPx deficiency. Synthesis of bile acids is regulated by negative feedback via their interaction with the farnesoid X receptor (FXR) [26]. Activated FXR in liver decreases expression of the rate-limiting enzyme in bile acid synthesis, cholesterol 7α-hydroxylase (CYP7A1). In the intestine, activated FXR induces synthesis of fibroblast growth factor 19 (FGF19), which also represses CYP7A1 expression. As for many other peroxisomal metabolic processes, it was the observation of bile acid abnormalities in patients with peroxisome biogenesis disorders that helped elucidate the complete bile acid biosynthetic pathway.
A role for peroxisomes in the synthesis of cholesterol and other isoprenoids from acetyl-CoA has been debated. Mass isotopomer and radioactive tracer studies are consistent with the notion that acetyl-CoA derived from peroxisomal VLCFA β-oxidation can be channeled toward cholesterol without mixing with the cytoplasmic acetyl-CoA pool [27, 28]. Cytoplasmic acetyl-CoA derived from glucose metabolism can also be used to synthesize cholesterol, suggesting dual subcellular localization of some early enzymes in the pathway. More controversial has been the localization of steps from mevalonate to farnesyl pyrophosphate (FPP). However, mevalonate kinase (MVK), phosphomevalonate kinase (PMVK), and mevalonate pyrophosphate decarboxylase (MVD) have now convincingly been shown to be cytosolic enzymes [29–31] suggesting that the pathway depicted in Figure 37.2 is accurate. Thus, mevalonate kinase (MVK) deficiency should no longer be considered a peroxisomal disease. The moderate reduction of plasma cholesterol observed in ZSD patients must have a different source.
Racemization of Pristanic and Bile Acids
Stereoisomers of certain substrates and intermediates in the pathways of branched-chain fatty acid degradation and bile acid synthesis are found in nature. These include phytanic acid, pristanic acid, bile acid precursors, and other intermediates that contain asymmetric carbon centers [32]. The α-oxidation of phytanic acid produces both 2 R– and 2S-stereoisomers of pristanic acid; however, ACOX2 can only utilize 2S-isomers as substrates for β-oxidation [33]. These two stereoisomers of pristanic acid can be interconverted by AMACR, an enzyme found in both peroxisomes and mitochondria by virtue of it’s having both a peroxisome and mitochondria targeting signal. The methyl groups on carbons 6 and 10 of pristanic acid are also in the R-configuration, and thus require AMACR as this fatty acid is progressively shortened via several cycles of β-oxidation. The bile acid precursors THCA and DHCA are also normally found as both R– and S-stereoisomers. Similar to the situation that exists with pristanic acid, only the 25S-stereoisomers of THCA or DHCA are substrates for ACOX2 and can thus be converted into mature bile acids [33]. AMACR converts 25 R– to 25S, thus facilitating utilization of the R-isomers. Patients with AMACR deficiency have elevated levels of 25 R bile acid precursors as well as 2 R-pristanic acid.
Ether Lipid Biosynthesis
Glycerophospholipids containing an ether-linked alkyl or alkenyl chain on the first carbon of glycerol instead of an ester-linked acyl group account for about 20% of membrane phospholipids and are further enriched in certain tissues and in specialized membranes, such as myelin and cardiac sarcolemma [34]. Most are alkenyl-containing ether lipids also known as plasmalogens. Peroxisomes contain three enzymes vital to initiating ether lipid synthesis: acyl-CoA reductase (FAR1; FAR2), dihydroxyacetone (glycerone) phosphate acyltransferase (GNPAT) and alkylglycerone phosphate synthase (AGPS) (Figure 37.2). The first step is the acylation of dihydroxyacetone phosphate (DHAP) at the first carbon position by GNPAT, transfer of acyl-DHAP across the enzyme active sites, followed by the exchange of the acyl group (fatty acid) for an alkyl group (fatty alcohol) by AGPS [35]. The alkyl group is provided by FAR1/FAR2. At this point, the ether-linked alkyl group is in place and further modifications to form mature plasmalogens take place in the endoplasmic reticulum [35].
The importance of ether phospholipids is highlighted by the severity of clinical symptoms in rhizomelic chondrodysplasia punctata (RCDP) [20]. Children with RCDP have deficient ether lipid synthesis resulting in shortening of proximal limbs (rhizomelia), punctate epiphyseal calcifications in long bones and profound growth restriction and intellectual deficiency. RCDP type 1 is caused by defects in PEX7, the PTS2 receptor. Because PEX7 is necessary for the import of PTS2 targeted peroxisomal matrix proteins, patients with this disorder are considered to have a peroxisome biogenesis disorder termed RCDP type 1. The known PTS2 targeted enzymes are AGPS, PHYH and ACAA1. Patients with RCDP1 have reduced plasmalogens and accumulate phytanic acid in tissues if it is included in the diet. Fatty acid β-oxidation is not affected, presumably because SCPx, which is targeted by the PEX5-PTS1 pathway, can take over the function of ACAA1 [36]. Thus, the pathophysiology of RCDP is mainly consequent to reduced tissue plasmalogen levels. Other isolated peroxisomal enzyme deficiencies causing plasmalogen deficiency and RCDP are listed in Table 37.1. Patients with RCDP of any type do not have liver disease.
Amino Acid Metabolism
Peroxisomes contain several important enzymes of amino acid metabolism, particularly alanine:glyoxylate transaminase (AGXT) and L-pipecolate oxidase (PIPOX). AGXT is found only in the liver and catalyzes the transfer of the α-amino group of alanine to glyoxylate, yielding glycine and pyruvate as products (Figure 37.2). AGXT removes glyoxylate produced by two other peroxisomal enzymes, hydroxyacid oxidase (glycolate oxidase) 1 (HAO1) and D-amino acid oxidase (DAO). These two enzymes, which convert glycolate and glycine, respectively, to glyoxylate, are typical flavin-linked peroxisomal oxidases that utilize molecular oxygen and produce H2O2. If not efficiently detoxified by the AGXT reaction, glyoxylate is further metabolized by glycolate oxidase to oxalate. AGXT is deficient in primary hyperoxaluria type I (PH1), in which accumulation of oxalate leads to the formation of calcium oxalate kidney stones and renal failure [37]. A common polymorphism near the amino terminus of AGXT creates a cryptic mitochondrial targeting signal that is unmasked by the presence of certain pathogenic variants in cis. This results in efficient mislocalization of AGXT to the mitochondria where it is unable to detoxify glyoxylate. While there is no direct liver involvement in patients with PH1, this disease may come to the attention of the hepatologist because of the need for a therapeutic combined kidney/liver transplant. AGXT is also deficient in peroxisome biogenesis disorders resulting in the development of calcium oxalate kidney stones in a subset of PBD-ZSD patients [38].
L-Pipecolic acid is synthesized in humans as an intermediate in the minor pathway of lysine catabolism. The peroxisomal enzyme L-pipecolic acid oxidase (PIPOX) is required for conversion of the imino acid pipecolic acid to Δ1-piperideine-6-carboxylic acid. The latter compound is converted to α-aminoadipic acid and ultimately to glutaric acid. Like other peroxisomal oxidases, PIPOX requires FAD and produces H2O2. Accumulation of L-pipecolic acid occurs in patients with PBD-ZSD, who were initially described as having hyperpipecolic acidemia (HPA) [39]. Mild elevations in plasma pipecolic acid are sometimes seen in DBP deficiency and Refsum disease. In addition, pipecolic acid levels are elevated in the mitochondrial disorder glutaric acidemia type 2 [40]. Finally, pipecolic acid is elevated and a biomarker for the nonperoxisomal disease, Vitamin B6 dependent seizures due to Antiquitin deficiency [41]. Only about 1% of lysine is degraded via pipecolic acid in the liver. A significantly greater proportion of lysine may be catabolized by this pathway in brain, and neurotoxicity of L-pipecolate has been suggested as contributing to the neurological problems in peroxisomal diseases [41].
Peroxide Degradation
Catalase (CAT) is the most abundant enzyme in peroxisomes – and one of the most abundant proteins in liver [2]. This enzyme plays a vital role in peroxisomal metabolism by decomposing H2O2 generated by peroxisomal oxidases (Figure 37.2). The catalase reaction proceeds via two mechanisms: a “catalatic” process (“C” in Figure 37.2) in which H2O2 is degraded to water and oxygen, and a “peroxidatic” process (“P” in Figure 37.2) in which a cosubstrate is oxidized by H2O2. Unlike many peroxisomal enzymes, catalase remains active in the cytosol in the absence of functional peroxisomes. Individuals who lack catalase (acatalasemia) are known, but are either asymptomatic or have ulcerating oral lesions that are often gangrenous.
In addition to catalase, other antioxidant enzymes such as superoxide dismutase reside in peroxisomes [42]. The role of this organelle in redox biology and antioxidant defense has only recently become appreciated. Current research efforts are aimed at investigating the consequences of peroxisome dysfunction on reactive oxygen and reactive nitrogen species, and at identifying downstream targets of oxidants produced by peroxisomes. The relevance of communication between peroxisomes and other organelles via redox signaling in metabolic homeostasis is an increasingly important area of research [42].
Biochemical Assays of Peroxisome Metabolism and Molecular Diagnostic Tools
The three biochemical pathways which are most useful in the laboratory diagnosis of peroxisomal diseases are the β-oxidation of VLCFA, α-oxidation of phytanic acid and biosynthesis of plasmalogens [43, 44] (see Table 37.2 for laboratory testing in peroxisomal disorders). Initial screening tests that target these metabolic pathways are capable of detecting most patients with peroxisomal diseases, and include measurement of plasma VLCFA, phytanic acid and erythrocyte membrane plasmalogen levels. Peduto et al. [45] also emphasized that elevations in plasma L-pipecolic acid can raise suspicion of a peroxisomal disorder. If a peroxisome biogenesis disorder is suspected on clinical grounds, quantitation of plasma VLCFA levels is the most informative initial biochemical assay (Table 37.2). In some laboratories, phytanic and pristanic acids can be quantitated in the same analysis, although these may not be informative based on diet and age of the patient. Peroxisome metabolites are measured by GC/MS and, more recently, by liquid chromatography-tandem mass spectrometry (LC/MSMS) [46]. Typically C26:0 is elevated while the amount of C22:0 is lowered resulting in a characteristic increase in C26/C22 and C24/C22 ratios. In peroxisomal disorders with liver involvement, the characteristic bile acid profile is elevation of C27 bile acids and reduction in C24 bile acids in serum and urine, which can be quantitated by LC/MSMS. C29 dicarboxylic acid, formed by chain elongation of THC-CoA, can also be detected in blood [47]. Skin biopsy for fibroblast culture is used for peroxisomal enzyme assays, subcellular distribution of catalase and immunofluorescence and/or immunoblot analysis for peroxisomal proteins/enzymes which can facilitate diagnosis in more difficult cases. Catalase is the enzyme most sensitive to peroxisome import deficiency and assessing whether catalase is contained within organelles or free in the cytoplasm is useful for diagnosis of milder forms of PBD-ZSD.
Moser and co-workers developed an LC/MSMS method for newborn screening for X-linked adrenoleukodystrophy using dried blood spots [48]. This assay measures the VLCFA content (as hexacosanoic acid, C26:0) of lysophosphatidylcholine with a high degree of sensitivity and accuracy. Newborn screening for X-linked adrenoleukodsytrophy (which also detects PBD-ZSD and other peroxisome single enzyme/protein disorders with elevations of VLCFA) was recently added to the US Recommended Uniform Screening Panel and thus a large number of these patients will soon be diagnosed in this fashion [49].
Molecular genetic testing approaches for peroxisomal disorders currently include a combination of gene-targeted testing (multigene panel) or whole exome sequencing using next generation sequencing technologies. Comprehensive peroxisome gene panels are available, as well as panels for cholestasis or Usher syndrome, which typically include many peroxisomal genes. It is not unusual for next generation sequencing approaches to be used early on in the diagnostic evaluations, with a return to peroxisome function testing to validate the gene variants detected, determine the extent of disease and for follow-up evaluations [50].
Skin fibroblast cultures can also be examined on a research basis to include localization of peroxisomal enzymes, as well as peroxisome numbers and morphology. These evaluations have generally shown reduced numbers of enlarged peroxisomes that are deficient in enzyme import in most patients with PBD-ZSD, ACOX1 or DPB deficiency [50]. PBD-ZSD patients with severe disease either lack peroxisomes, or show aberrant peroxisome structures devoid of peroxisomal enzymes (ghosts), whereas milder disease is associated with increased peroxisome numbers with maintenance capacity of some degree of enzyme import. Other observations in fibroblasts of patients with milder clinical phenotypes include “peroxisomal mosaicism,” which describes cells with import competent peroxisomes observed by punctate staining of a marker enzyme, adjacent to cells in which marker enzymes have a cytosolic location [20]. In individuals with the mildest phenotypes, cultured skin fibroblasts can show normal peroxisome numbers and function. In some of these cases, culturing patient fibroblasts at 40°C exacerbated the aberrant peroxisome phenotypes by causing a complete absence of catalase-containing peroxisomes and thus assisting in diagnosis [20]. Clinical severity of disease can also be generally correlated to plasma levels of VLCFA and plasmalogens, with highest VLCFA and most deficient plasmalogens in severe patients, normal plasmalogens in milder patients and generally normal overall peroxisome functions in the mildest patients. Other observations include fluctuations in peroxisome metabolites when followed over time, as well as improvement or even normalization of peroxisome metabolites in adulthood [51].
Peroxisome Disorders with Liver Involvement
Peroxisome Biogenesis Disorders: Zellweger Spectrum Disorders
The Zellweger spectrum is a heterogeneous disorder primarily due to biallelic mutations in any one of 13 PEX genes (PEX1, PEX2, PEX3, PEX5, PEX6, PEX10, PEX 11β PEX12, PEX13, PEX14, PEX16, PEX19, and PEX26). Recently, allelic expression imbalance was shown to promote a dominant expression for heterozygous mutations in PEX6 [52]. These disorders are pan-ethnic and the overall estimated birth incidence is 1/50,000 [20]. The phenotypic spectrum is broad and includes patients with symptoms ranging from mild to severe. The use of historical terms such as “infantile Refsum disease” (mild), “neonatal adrenoleukodystrophy” (intermediate), and “Zellweger syndrome” (severe) is now discouraged. The current term, Zellweger Spectrum Disorder (ZSD), embraces the common peroxisome etiology and allows for inclusion of the atypical and novel phenotypes now being recognized with next generation sequencing techniques. The severity of the clinical picture is dictated by the effect of the mutation on PEX protein function, with severe disease generally associated to absent or nonfunctional PEX proteins, and milder disease associated to PEX proteins with residual functions.
There are several founder alleles that account for the high frequency of PEX1 c.2528A>G allele (p.Gly843Asp) and PEX1 c.2097_2098insT allele (p.Ile700Tyrfs42*) in persons of Northern European heritage [53], PEX10 c.814_815del CT in Japan [54] and PEX6 c.802_815del in French Canadians [55]. In North America, mutations in PEX1 account for around 60–70%, with other genes each accounting for 10% or less of all PBD-ZSD patients [56]. Given the high frequency of the PEX1 founder alleles in North America and Europe, there are a relatively large number of patients with these alleles. Clinical observations have generally shown that PEX1 p.Gly843Asp homozygotes have milder ZSD phenotypes, PEX1 p.G843D/Ile700fs compound heterozygotes have intermediate phenotypes and PEX1 p. Ile700fs homozygotes have severe phenotypes.
Clinical Manifestations of Severe Peroxisome Biogenesis Disorder-Zellweger Spectrum Disorder
Severe ZSD is a classic congenital malformation syndrome originally described by the pediatrician Hans Zellweger [57], and subsequently referred to by its characteristic features as Zellweger cerebro-hepato-renal syndrome [58–60]. Thus, the most severe degree of peroxisome dysfunction affects fetal organ development. Patients present as newborns with severe hypotonia, seizures and characteristic craniofacial dysmorphology including wide anterior fontanelle with delayed closure, a tall forehead, underdeveloped supraorbital ridges, broad nasal bridge, epicanthal folds, anteverted nostrils and micrognathia. These infants are born with major malformations of the brain, kidney and skeleton. Brain MRI reveals abnormal cortical gyral patterns consequent to neuronal migration defects, with a characteristic pattern of lateral (perisylvian) microgyria and median (perirolandic and occipital) pachygyria; germinolytic cysts in the caudothalamic groove are also present [61]. Renal ultrasound shows multiple small renal cortical cysts and skeletal X-rays often show chondrodysplasia punctata at the hips and knees. Hepatomegaly and liver dysfunction are present. Eye disease includes cataracts, congenital glaucoma, retinal dystrophy and optic atrophy. Sensorineural hearing loss is present. Hypotonia and poor feeding is persistent, often with aspirations. Most infants with severe ZSD die within the first year of life, and virtually all infants by two years of age, usually due to neurological compromise.
Intermediate and Milder Peroxisome Biogenesis Disorder-Zellweger Spectrum Disorder
The majority of patients with PBD-ZSD have intermediate and milder phenotypes, with longer survival and improved neurological outcomes. Overall this group is distinguished from severe ZSD by the absence of congenital malformations and instead a progressive disorder of peroxisome dysfunction over time [51]. Renal cysts, chondrodysplasia punctata and classic brain gyral abnormalities are not observed, although subtle small areas of polymicrogyria are infrequently reported in intermediate ZSD. The core phenotype involves progressive sensorineural hearing loss and retinal dystrophy, often leading to their classification as deaf-blind children, Usher syndrome or Leber hereditary optic neuropathy. There is also neurocognitive delay, liver abnormalities and amelogenesis imperfecta of the secondary teeth. The characteristic facial dysmorphic features observed in severe ZSD are attenuated or absent. Minor physical anomalies include cryptorchidism, pilonidal dimple and single transverse palmar creases. Height and weight parameters are typically at the lower end of the normal population growth curves, whereas head circumferences are usually normal for age.
These individuals are at risk to develop additional secondary complications, including adrenal insufficiency [62], seizure disorders, leukodystrophy [63], peripheral neuropathy, cerebellar atrophy, liver fibrosis, renal stones [38] and osteopenia [64]. The prediction of which children will develop these complications and when, as well as the timeline of their progression remains unknown. Generally, patients with intermediate phenotypes have more rapid deterioration of vision and hearing, and develop multi-system complications earlier on in life than patients with milder phenotypes. Their survival is variable from early childhood through adulthood. Patients with milder ZSD phenotypes often survive through adulthood. These individuals can have normal intellect and are often recognized by the triad of sensorineural hearing loss, retinal pigmentary degeneration and amelogenesis imperfecta [65, 66]. It is likely that genetic and environmental modifiers also contribute to phenotype complexity considering that within the mild and intermediate severity groups there is still a wide range of disease manifestations, including variability between unrelated patients with the same genotypes and variability in outcomes within sibships [67]. Clinical features of ZSD by severity and age of onset, and general guidelines for patient management and care were reported [44, 68].
Variant phenotypes in children and adults were recently reported and feature progressive cerebellar ataxia and peripheral neuropathy with relative preservation of intellect and without major sensory involvement. These atypical phenotypes were associated with specific mutations in the RING zinc finger domains of PEX2, 10, 12 [69–71]. Specific mutations in the C-terminal region of PEX16 are also associated with this atypical phenotype, but also features progressive spastic paraparesis, leukodystrophy, dystonia and juvenile cataracts [72, 73]. Some of these patients have had liver disease [74, 75], but retinal pigmentary degeneration and sensorineural hearing losses have not been reported.
Liver Disease in Peroxisome Biogenesis Disorder-Zellweger Spectrum Disorder
Clinical Evolutions and Liver Histology
We reviewed descriptive case reports on liver disease in ZSD over the past 50 years. These reports are summarized below. Hepatic abnormalities observed in ZSD, as well as isolated peroxisomal protein/enzyme deficiencies, are listed in Table 37.3.
Table 37.3 Hepatic Abnormalities Identified in Peroxisomal Disorders
Disorders | Clinical Features | Hepatic Symptoms | Liver Functions | |||||||||||||||
---|---|---|---|---|---|---|---|---|---|---|---|---|---|---|---|---|---|---|
Hepatomegaly | Jaundice | Liver Failure | Cholestasis | Hepatitis | Steatosis | Fibrosis | Cirrhosis | Liver Tumor | HCC | AST | ALT | ALP | PT | INR | Direct Bilirubin | Cholesterol | Triglyceride | |
ZSD (severe) | x | x | x | x | x | x | x | ↑ | ↑ | ↑ | ↑ | ↑ | ↑ | |||||
ZSD (intermediate–mild) | x | x | x | x | x | x | x | x | x | x | ↑ | ↑ | ↑ | ↑ | ↑ | ↑ | ↓ | ↓ |
1Acyl-CoA oxidase 1 deficiency | x | x | ↑ | ↑ | *↑ | |||||||||||||
Acyl-CoA oxidase 2 deficiency | x | ↑ | ↑ | ↑ | *NL | 2↑- NL | *↓ | |||||||||||
1D-Bifunctional protein deficiency | x | x | x | x | x | 2NL | 2↑- NL | 2NL | 2NL | ↑ | *↑ | |||||||
1AMACR deficiency | x | x | * | x | 2↑ | 2↑ | 2NL | ↑ | ↑ | 2↑- NL | ||||||||
*ABCD3 Deficiency | x | x | x | x | x | ↑ | ↑ | ↑ | ↑ | ↑ | ↑ | |||||||
BAAT deficiency | x | x | * | x | * | * | x | ↑ | ↑ | ↑ | ↑ | ↑ | ↑ |
x indicates presence of finding, blank indicates finding not reported, ↑ indicates elevation, ↓ indicates reduction, * indicates only one patient has been described.
1 Milder forms of ACOX1, DBP and AMACR may not have any liver disease. NL indicates normal, ↑- NL indicates elevated or normal.
2 Liver function test were only reported in a few patients.
In a second trimester fetus with severe ZSD, the liver was structurally normal although liver peroxisomes were absent [76] suggesting that liver disease develops prominently in postnatal life secondary to peroxisome dysfunction. Infants with severe ZSD experience transient neonatal jaundice and hepatobiliary cholestasis, accompanied with conjugated hyperbilirubinemia in early infancy. While liver ultrasonography may be normal at birth, liver functions are abnormal and liver disease can progress rapidly into fibrosis and cirrhosis, and infrequently, to liver failure within the first year of life [77, 78]. Review of 34 patients with severe ZSD [59, 60, 77–87] showed enlarged and firm livers with a micronodular surface by 20 weeks of age. In 16 of 34 patients, liver from biopsy and autopsy samples frequently revealed abnormal hepatic lobular architecture represented by an irregular spatial relationship between the central vein and peripheral portion of the lobule. Regular liver plates were less numerous or absent. Crowded assembly of central veins was observed without intervening portal tracts, while in other areas, abnormally scattered central veins were seen. Loss of bile ducts were noted. In 23 of 34 patients, portal fibrosis or intralobular fibrosis of various degrees was observed, with a few culminating in micronodular cirrhosis. Frequently observed findings were lobular disarray, inflammatory cell infiltration, foci of hepatocytes necrosis, bile pigments in hepatocytes or bile canaliculi and liver macrophage (Kupffer cells) siderosis in cytoplasm or lysosomes. Huybrechts et al. [87] reported a child with jaundice and hepatosplenomegaly in which a liver biopsy at age three months was notable in showing bile duct paucity, cholestasis, and arterial hyperplasia, resembling Alagille syndrome. Excess iron deposition and elevated serum iron have been observed in young ZSD patients usually in Kupffer cells, but sometimes in hepatocytes [83]. Its presence was not correlated to liver disease progression [88].
Review of 37 patients with intermediate-mild ZSD spectrum [81, 89–101] showed transient neonatal jaundice caused by cholestasis in eight patients. Hepatomegaly was observed in 29 patients as a feature that was either slight and inconsistent, or marked and persistent. Clinical manifestations of liver disease were generally absent, but worsened in some individuals with time. These progressions included fibrosis, steatosis, portal hypertension, esophageal varices, bleeding episodes, and ascites as complications. In the vast majority of intermediate-mild patients reviewed, the initial ultrasound examinations reported normal liver structure. Biopsies done in the first year of life showed enlarged hepatocytes with intracanalicular or intrahepatocellular cholestasis. Foci of single hepatocyte necrosis, giant cell transformation, pseudoacinar transformation and increased mitotic activity were occasionally identified also at an early age. Other infrequent findings included hepatocyte intranuclear glycogen inclusions, prominent nucleoli, abundant secondary lysosomes and micro- or macrovacuolated cytoplasm consisting of lipid droplets. Compared to severe ZSD patients, liver fibrosis was usually mild or absent in this group of patients (26 of 37), but developed with age culminating in cirrhosis (seven of 37). Portal inflammatory infiltrates were recognized in 12 out of 37 patients. In patients with severe liver disease, regenerative nodules accompanied by bridging fibrosis were noted.
Thirteen patients within this intermediate-mild ZSD group were divided into two severity classes based on liver-related symptoms [95]. The first group (five patients) demonstrated early onset of significant liver disease evident by hepatomegaly, prolonged neonatal jaundice, coagulopathy and liver fibrosis, and two of these individuals developed subsequent liver failure. In one patient, serial assessment identified liver cirrhosis at age eight years. She died at age 18 years due to liver failure with complications. Autopsy showed extensive cirrhosis with prominent dysplastic nodules in the setting of portal hypertension and ascites. In the second group (eight patients), although hepatic manifestations such as hepatomegaly, neonatal jaundice and coagulopathy were infrequently observed, the liver histology (from biopsy and autopsy samples) also revealed silent fibrosis (seven of eight) progressing to bridging fibrosis (six of eight). Among these patients, one of them had mild lobular inflammation and periportal fibrosis on liver biopsy at age six months. At age ten years, she developed liver cirrhosis with multiple regenerative nodules visualized by MRI. Another patient without any symptoms or signs of liver disease during her lifespan showed cirrhosis and poorly differentiated hepatocellular carcinoma on autopsy at age 36 years, after death from respiratory failure.
Serial biopsies were reported in five additional intermediate-mild patients [89, 94, 98, 102]. Warren et al. [94] provided an analysis of liver disease progression in a patient who had neonatal jaundice and hepatosplenomegaly. Liver biopsy performed at age five months for diagnosis revealed cholestasis, mild steatosis, scattered hepatocyte necrosis, minimal portal fibrous expansion and mild portal inflammation. Liver ultrastructure showed almost complete loss of peroxisomes by catalase staining, dilated endoplasmic reticulum and possible early trilamellar inclusions in fat droplets within hepatocytes. Liver biopsy was done for persistent hepatomegaly at age 14 years and indicated advanced liver disease with thick bridging fibrosis, minimal inflammation and occasional micronodules. Liver ultrastructure showed abundant trilamellar structures and lipid droplets within large angulated lysosomes in both hepatocytes and Kupffer cells. Some hepatocytes showed abnormal mitochondria with electron-dense matrix and cystic dilation of cristae. Chow et al. [89] report a liver biopsy done for diagnosis at age six months which showed moderate fibrosis and occasional macrophages at portal tracts. Liver histology at autopsy at age 3.5 years revealed well-established cirrhosis and prominent macrophage accumulation.
Heubi et al. [98] report serial liver biopsies on two intermediate-mild patients before and after oral cholic acid supplementation. The first patient had a liver biopsy at age seven months that showed lobular disarray, pseudoacinar transformation, focal mild periportal fibrosis and minimal inflammation. Focal cell necrosis and hepatocyte proliferation were occasionally seen. Cholic acid supplementation began at age 13 months and liver biopsy at age 20 months showed unchanged histopathology. Biopsy from the second patient at age three months showed bridging fibrosis with giant cell formation. At age 16 months there was persistent bridging fibrosis with periportal inflammation and lobular cholestasis. At age three years, liver histology showed mosaic hepatocyte architecture and infrequent pseudoacinar transformation. After a year of cholic acid supplementation, liver histology remained stable. In contrast, long-term cholic acid supplementation in another patient did not prevent liver disease progression [102]. In this individual, liver biopsy at age four months revealed cholestasis, necrosis, giant cell formation, periportal inflammation and moderate fibrosis. Liver histology one year later showed similar findings, except for reduced cholestasis and enhanced bridging fibrosis. After five months of cholic acid supplementation, liver biopsy showed improvement of inflammation without further progression of fibrosis. However, he subsequently developed cirrhosis and portal hypertension and at age 19 years he developed hepatocellular cancer.
These emerging reports indicate that, although the majority of intermediate-mild ZSD patients do not exhibit clinical symptoms of liver disease, a small number are at risk for a progressive cirrhosis that can lead to liver failure and hepatic cancer. Data from our “Longitudinal Retrospective Natural History Study of Patients with Peroxisome Biogenesis Disorders (PBD)” (ClinicalTrials.gov Identifier: NCT01668186) supports these reports. In this cohort, 63 patients with ZSD were reviewed. Of 52 mild-intermediate patients, the initial liver ultrasound examination frequently showed normal architecture. However, subsequent ultrasonography revealed coarse, heterogeneous liver echotexture (12 of 52) and altered parenchymal echogenicity (two of 52). Hypoechogenic masses (four of 52) and multiple hyperechogenic foci were occasionally reported (two of 52). In one 23-year-old patient, ultrasonography identified liver fibrosis and cirrhosis with hypodense lesions. At age 25 years he developed steatosis and metastatic liver cancer. In a mild ZSD patient who remained clinically asymptomatic in earlier years of life, abdominal ultrasound at age 43 years revealed heterogeneous echotexture, numerous hyperechogenic lesions and hepatic hemangioma. In a third patient, the liver contained several hyperechogenic and hypoechogenic masses which ruptured causing hemoperitoneum surrounding the liver and spleen at age 19 years. Liver function tests obtained in this cohort showed elevations of alanine transaminase (ALT), aspartate transaminase (AST), and alkaline phosphatase (ALP). In this population, liver functions were elevated in the first one to two years of life and then generally decreased to one-to two times the upper limit of normal ranges (Figure 37.3). Although fewer reports of C27 bile acids were available, these also showed a similar pattern. Gamma-glutamyl transpeptidase (GGT) and albumin usually stayed within normal ranges. Prolonged prothrombin time (PT) and higher international normalized ratio (INR) were observed. Malabsorption of vitamin K caused coagulopathy and rickets was reported due to malabsorption of vitamin D.
Figure 37.3 Liver functions in a ZSD cohort. In the NCT01668186 cohort, levels of alkaline phosphatase (ALP), alanine aminotransferase (ALT), aspartate aminotransferase (AST), and dihydroxycholestanoic acid (DHCA) decrease with age. Shown are plasma ALP, ALT, and AST levels for all patients across ages 0–45 years and plasma DHCA level for all patients across ages 0–43 years. For ALP: 60 intermediate and mild ZSD patients (427 data points in black) and two severe patients (16 data points in red) are presented. For ALT: 67 intermediate and mild ZSD patients (500 data points in black) and two severe patients (14 data points in red) are presented. For AST: 54 intermediate and mild ZSD patients (346 data points in black [3 additional points > 1,200 U/L and not presented] and five severe patients (28 data points in red) are presented. For DHCA, representing C27 bile acids, 33 intermediate and mild ZSD patients (41 data points in black). Among these 33 patients, five received cholic acid (CA) at the time of sampling (7 data points in green). The reference upper limit of normal is shown with the blue line. Note that these values do not reflect serial measurements on every individual.
Liver Ultrastructure
Reports of transmission electron microscopy (TEM) of liver in ZSD patients is limited, but has generally shown decreased or absent hepatic peroxisomes across severity groups visualized by staining for catalase or immunogold labeling of other matrix enzymes. Limitations of these techniques include using matrix enzyme markers to document peroxisome structure rather than markers for peroxisomal membrane proteins, which would allow accurate visualization of residual peroxisomal structures. Furthermore, catalase import is the most sensitive to peroxisome dysfunction and can be disrupted in milder disease when import of other matrix enzymes is preserved.
Liver peroxisomes in ZSD patients may contain an electron-dense core absent in normal human hepatocytes, and membrane invaginations which enclose glycogen or iron particles [90, 103]. Many hepatocytes contain numerous phagolysosomes, in which iron deposits may be found, cytoplasmic micro- or macrovacuolated lipid droplets and decreased or abnormally distributed glycogen rosettes [91, 104]. Hepatocyte mosaicism for peroxisome content has been observed by ultrastructure, as well as light microscopy [75]. Mitochondria in most hepatocytes are normal. However, in damaged hepatocytes with abundant lysosomes, mitochondria are observed with increased electron-dense matrix, distortion of cristae, large size and crystalline inclusions [94, 105]. Intrahepatic bile ducts were reported as normal, deficient, or hyperplastic. In one patient there was dilated bile canaliculi with short villi and excess free ribosomes [82]. Macrophages contained fusiform or angulated phagolysosomes with lipid droplets and trilamellar crystalline inclusions. The crystalline structure consisted of two or three needle-shape, parallel-stacked lamellae that are regularly spaced, insoluble in acetone and xylene, and strongly birefringent under polarized light [106]. Trilamellar inclusions are more abundant in Kupffer cells than in hepatocytes. The amount of trilamellar inclusions increases with age, but can be observed in infancy in severe ZSD patients [106].
Single Peroxisomal Protein/Enzyme Deficiencies Associated with Liver Disease
Distinction between patients with isolated deficiencies of ACOX1, DBP and other single protein defects and those with ZSD can often be clinically challenging. Biochemical and molecular analyses help to facilitate a correct diagnosis.
ABCD3 Deficiency
ABCD3 transports methyl-branched chain fatty acids and bile acids into the peroxisome for subsequent oxidation. It is one of the most abundant peroxisome membrane proteins in hepatocytes. ABCD3 deficiency was reported in a single patient by Ferdinandusse et al. [24]. This patient presented with mild transient jaundice during infancy but with normal growth and development. At age 1.5 years, she developed severe liver disease characterized by hepatosplenomegaly, severe anemia, elevated serum transaminases, hyperbilirubinemia and coagulopathy. Liver biopsy showed minimal inflammatory infiltrates, hepatocyte regeneration and fibrosis. At age three years, she developed cholestasis with portal hypertension, umbilical collaterals and esophageal varices. At age four years, liver transplantation was performed for decompensated liver cirrhosis with hepatopulmonary syndrome. Liver explant showed micronodular cirrhosis. Peroxisome functions showed marked accumulation of plasma C27 bile acid intermediates, C29 dicarboxylic acid and VLCFA, with normal erythrocyte plasmalogen levels. Although phytanic and pristanic acid levels were normal, this was likely dietary as β-oxidation in fibroblasts was reduced. Further investigative studies of peroxisome functions led to diagnosis.
Acyl-CoA Oxidase 1 (ACOX1) Deficiency
This disorder was initially termed pseudoneonatal adrenoleukodystrophy as the patients resembled those with intermediate ZSD [107]. Patients had neonatal hypotonia and seizures, severely delayed psychomotor function, retinal degeneration and optic atrophy, sensorineural hearing loss and early neurological regression consequent to central nervous system demyelination [107]. Some of these patients had cortical dysplasia. Dysmorphic facial features were recognized that resembled ZSD. The biochemical hallmark of this enzyme deficiency is an isolated increase in plasma VLCFA. Hepatomegaly was reported in half of the patients in a large cohort with ACOX1 deficiency [108]. Liver biopsy in one patient showed enlarged peroxisomes, and in another patient, liver fibrosis was documented on autopsy. Two patients reported by Carrozzo et al. [109] had elevated serum aminotransferases and in one patient, abdominal ultrasound showed diffuse liver hyperechogenicity at age one year. Urine organic acids showed dicarboxylic (C8-C10) and epoxydicarboxylic (C12-C14) aciduria. Not surprisingly, patients with milder phenotypes are now reported, making this disorder a clinical spectrum also. Ferdinandusse et al. [110] report siblings age 52 and 55 years with normal developmental milestones and neurological deterioration in childhood. They had retinal degeneration, cataract, and marked atrophy of brainstem and cerebellum; liver disease was not described. The milder clinical presentation correlated to residual ACOX1 enzyme activity in fibroblasts.
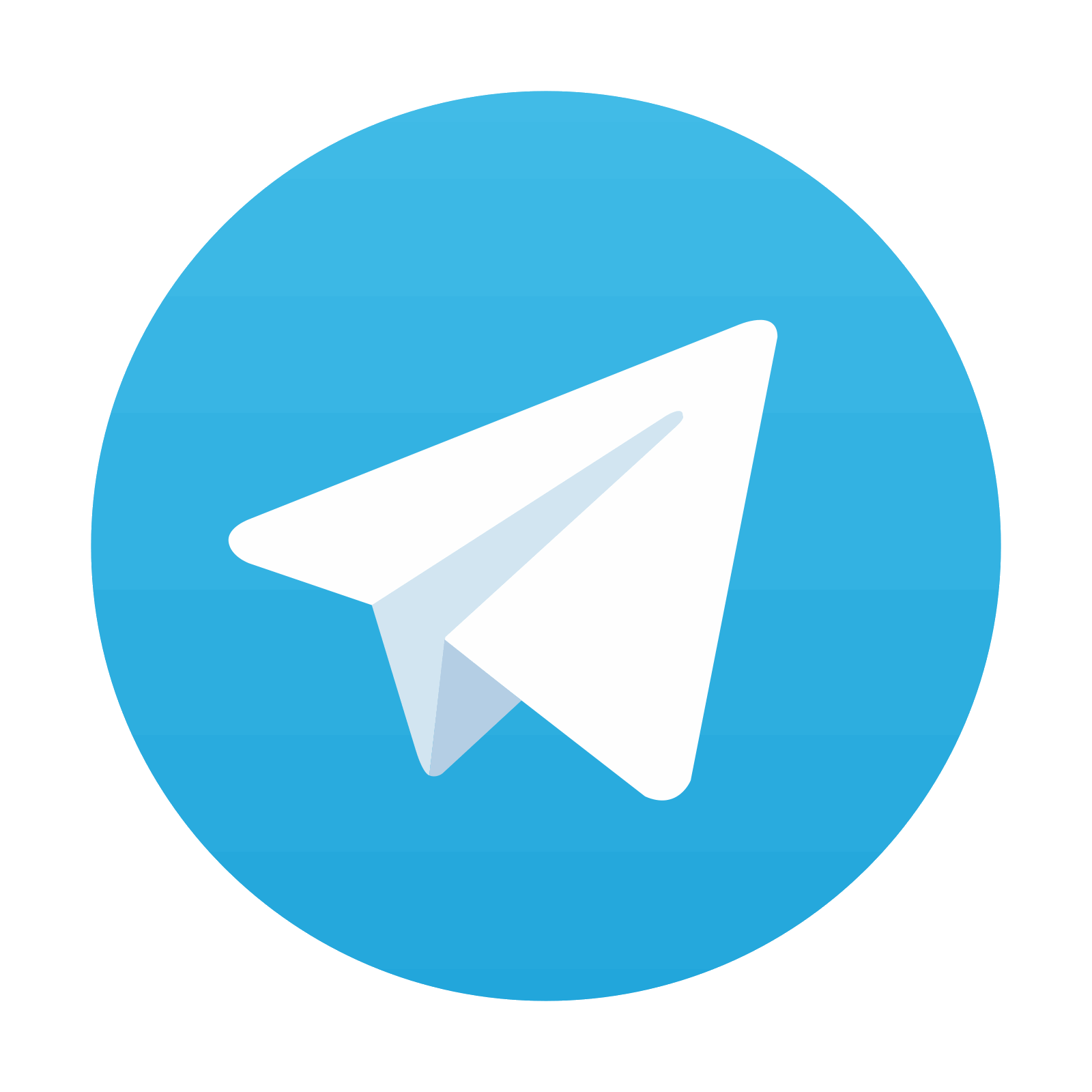
Stay updated, free articles. Join our Telegram channel
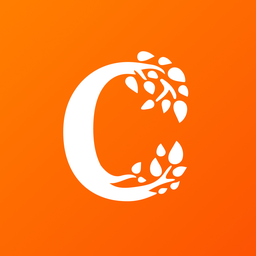
Full access? Get Clinical Tree
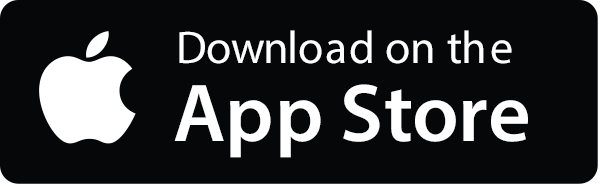
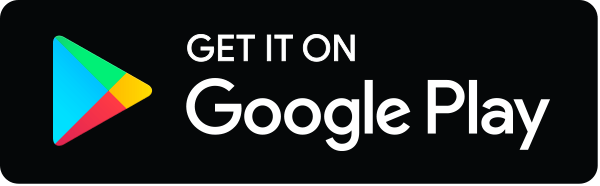
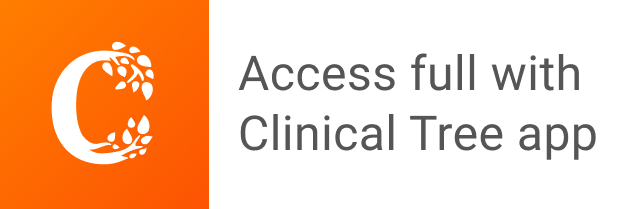