Abstract
Mitochondrial fatty acid β-oxidation (FAO) is an essential component of energy production and homeostasis in humans. During periods of limited glucose supply, FAO in the liver provides energy for hepatic function and the acetyl-CoA substrate needed for hepatocytes to synthesize and release ketone bodies into circulation. Ketone bodies provide an alternative energy substrate for peripheral tissues when glucose supply is limited. Other tissues such as skeletal and cardiac muscle rely on FAO for energy production. The oxidation of fatty acids can provide up to 80% of the energy requirements for cardiac and skeletal muscle while sparing glucose for use by the brain and CNS during moderate exercise, fasting, or illness. Disorders in the ability to use fatty acids for energy production manifest during periods of increased energy demands or reduced caloric intake.
Introduction
Mitochondrial fatty acid β-oxidation (FAO) is an essential component of energy production and homeostasis in humans. During periods of limited glucose supply, FAO in the liver provides energy for hepatic function and the acetyl-CoA substrate needed for hepatocytes to synthesize and release ketone bodies into circulation. Ketone bodies provide an alternative energy substrate for peripheral tissues when glucose supply is limited. Other tissues such as skeletal and cardiac muscle rely on FAO for energy production. The oxidation of fatty acids can provide up to 80% of the energy requirements for cardiac and skeletal muscle while sparing glucose for use by the brain and CNS during moderate exercise, fasting, or illness. Disorders in the ability to use fatty acids for energy production manifest during periods of increased energy demands or reduced caloric intake.
At least 22 different inherited genetic disorders in the mitochondrial FAO pathway have been described [1, 2]. Most of the disorders, divided into defects in long and medium chain substrates, have an increasingly broad range of recognized phenotypes. Severe phenotypes of both groups present in infancy with catastrophic episodes of fasting or illness-induced hypoketotic hypoglycemia and associated hyperammonemia. Prior to the introduction of expanded newborn screening they often went unrecognized until sudden unexpected death, with as many as one-third of the initial episodes being fatal in the first two to three years of life [3–5]. The most common clinical presentation of FAO disorders in early childhood includes nausea, vomiting, somnolence, and hepatic encephalopathy, similar to what was once known as Reye syndrome, that can progress to coma and death if untreated. Cardiomyopathy can be a life-threatening complication at any age in long chain FAO defects. Most patients with long chain FAO defects transition to a clinical picture dominated by recurrent rhabdomyolysis by five to six years of age. Additionally, mild phenotypes of FAO deficiency may present in adolescence or adulthood with exercise intolerance with recurrent episodes of rhabdomyolysis and myoglobinuria. Patients with milder phenotypes who present later in life typically previously have not reported episodes of hypoketotic hypoglycemia during fasting or illness [3].
The reported combined incidence of FAO disorders across Australia, Germany, and the USA is one in 9,300 live births [6, 7]. The incidence is lower among Asians, largely due to a decreased incidence of medium chain acyl-CoA dehydrogenase deficiency. All of the FAO disorders are inherited in an autosomal recessive manner. Specific gene defects have been identified for most of the disorders. Table 34.1 lists the enzymes in the FAO pathway that will be described in this chapter, their corresponding OMIM number, and gene location. This chapter will review the current knowledge in the field of FAO disorders with a specific focus on the liver phenotypes observed.
Enzyme | Deficiency Syndrome | OMIM Number | Gene, Location |
---|---|---|---|
Organic cation transporter 2 (OCTN2) | Primary carnitine deficiency/carnitine uptake defect | 603377 | SLC22A5, 5q31.1 |
Carnitine palmityltransferase-IA | Enzyme deficiency | 600528 | CPT1A, 11q13 |
Carnitine palmityltransferase-IB | Unknown | 601987 | CPT1B, 22qter |
Carnitine palmityltransferase-IC | Unknown | 608846 | CPT1 C, 19q13.33 |
Carnitine/acylcarnitine translocase | Enzyme deficiency | 212138 | SLC25A20, 3p21.31 |
Carnitine palmityltransferase-II | Enzyme deficiency | 600650 | CPT2, 1p32 |
Very-long-chain acyl-CoA dehydrogenase | Enzyme deficiency | 609575 | ACADVL, 17p13 |
Long-chain acyl-CoA dehydrogenase | Unknown | 201460 | ACADL, 2q34-q35 |
Mitochondrial trifunctional protein (TFP) | TFP deficiency, long-chain 3-hydroxyacyl-CoA dehydrogenase deficiency | 600890 (α-subunit) 143450 (β-subunit) | HADHA and HADHB, 2p23 |
Medium-chain acyl-CoA dehydrogenase | Enzyme deficiency | 607008 | ACADM, 1p31 |
Medium-chain 3-ketoacyl-CoA thiolase (T1) | Enzyme deficiency | 602199 | T1, 18q.21.1? |
Short-chain acyl-CoA dehydrogenase | Enzyme deficiency | 201470 | ACADS, 12q22-qter |
Short-chain enoyl-CoA hydratase (crotonase) | Unknown | 602292 | ECHS1, 10q26.2-q26.3 |
Medium/short-chain 3-hydroxyacyl-CoA dehydrogenase (3-hydroxyacyl-CoA dehydrogenase 1) | Enzyme deficiency | 300256 | Xp11.2 |
Short-chain 3-ketoacyl-CoA thiolase | Enzyme deficiency | 607809 | T2, 11q22.3-q23.1 |
(β-ketothiolase, T2) | |||
Electron transfer flavoprotein, α-polypeptide | Multiple acyl-CoA dehydrogenase deficiencies/glutaric aciduria IIA | 608053 | ETFA, 15q24.2-q24.3 |
Electron transfer flavoprotein, β-polypeptide | Multiple acyl-CoA dehydrogenase deficiencies/glutaric aciduria IIB | 130410 | ETFB, 19q13.41 |
Electron transfer flavoprotein dehydrogenase | Multiple acyl-CoA dehydrogenase deficiencies/glutaric aciduria IIC | 231675 | ETFDH, 4q32.1 |
Riboflavin transporter 1 | Riboflavin deficiency | 607883 | SLC52A1, 17p13.2 https://omim.org/geneMap/17/91?start=-3&limit=10&highlight=91 |
Riboflavin transporter 3 | Brown-Vialetto-Van Laere syndrome 2 | 607882 | SLC52A2, 8q24.3 |
Riboflavin transporter 2 | Brown-Vialetto-Van Laere syndrome 1 | 613350 | SLC52A3, 20p13 |
Mitochondrial folate carrier | Riboflavin responsive exercise intolerance | 610815 | SLC25A32, 8q22.3 |
Flavin adenine dinucleotide synthetase | Lipid storage myopathy | 610595 | FLAD1, 1q21.3 |
Regulation of Fatty Acid Oxidation
Figure 34.1 is a schematic of the FAO pathway and its interactions with other mitochondrial energy pathways. Substrate availability and hormonal regulation acutely regulate the pathway. In addition, cellular energy demands can affect FAO flux. Finally, long-term regulation by hormone transcription factors such as the PPAR family can also alter flux through the FAO pathway.
Figure 34.1 Fatty acid oxidation interacts with two other mitochondrial energy pathways, the electron transport chain and the tricarboxylic acid (TCA) cycle. FAO protein abbreviations are as defined in the text. TCA cycle abbreviations are as follows: AC-CoA: acetyl-CoA; CIT: citrate; ICIT: isocitrate; aKG: alpha-ketoglutarate; SUCC-CoA: succinyl-CoA; SUCC: succinate; FUM: fumarate; MAL: malate; OAA: oxaloacetate.
Most tissues can use a variety of substrates to produce energy depending on availability and physiologic state; skeletal muscle and liver can use glucose or fatty acids, and the CNS can use glucose or ketones. During post-prandial periods with abundant glucose supply, insulin is released from the pancreatic beta cell, increasing glucose uptake into muscle and adipose tissue and suppressing free fatty acid release from adipocytes. In this setting, the predominant substrate available and oxidized is glucose. Glycolysis raises the cytosolic concentration of malonyl-CoA, which allosterically inhibits carnitine palmitoyltransferase (CPT-IA), the first and rate-limiting step of FAO in most tissues [8].
During periods of fasting, glucose and insulin concentrations decrease, and free fatty acids are mobilized and released into the circulation. Free fatty acids are transported into the cell for oxidation via a variety of cellular fatty acid transporters. Intracellular malonyl-CoA concentrations decrease and release the inhibition of CPT-IA. Fatty acids enter the mitochondria via the carnitine transport system and are oxidized to produce reducing equivalents for the respiratory chain, ultimately leading to adenosine triphosphate (ATP) production. In the liver, acetyl-CoA from FAO is used to produce the ketone bodies, acetoacetate and β-hydroxybutyrate. In the fasting state, FAO is upregulated, and fatty acids and ketones become a primary energy substrate in some tissues.
Dietary macronutrient composition can also influence flux through the FAO pathway. High-fat diets increase FAO because many fatty acids are ligands for the peroxisome proliferator-activating receptor (PPAR) family of nuclear hormone transcription factors. Dietary fatty acids bind to PPAR and the ligand/transcription factor complex translocates to the nucleus. The PPARs bind nuclear receptor response elements in the promoter region of target genes. PPARβ–ligand binding especially enhances transcription and translation of most mitochondrial energy related genes, including those of FAO, the tricarboxylic acid (TCA) cycle, and oxidative phosphorylation (OXPHOS) [9].
Exercise increases FAO by increasing energy demands and mobilizing fatty acids for oxidation. Moderate intensity exercise increases hormones such as adrenaline, glucagon, and adrenocorticotropic hormone, increasing lipolysis of triglycerides in adipose tissue. Increased energy utilization depletes tissue glucose stores and lowers intracellular malonyl-CoA concentrations. Low malonyl-CoA concentrations increase CPT-IA activity and entry of fatty acids into the mitochondria for oxidation.
Fatty Acid Oxidation
Circulating free fatty acids or fatty acids released from lipoproteins by lipoprotein lipase are taken up into the cell via a variety of cell surface fatty acid transporters. Some of these transporters have endogenous acetyl-CoA synthetase activity and some do not. All fatty acids are rapidly esterified to acyl-CoAs via the transporter itself or by an acyl-CoA synthetase. From the cytosol, fatty acids must enter the mitochondria for oxidation. Medium- and short-chain fatty acids appear to diffuse into the mitochondria, but long-chain fatty acids are shuttled into the mitochondria by the carnitine palmitoyl-transport (CPT) system [2]. Carnitine itself is transported into cells by a specific high affinity transporter, the OCTN2 protein, but can also enter through a variety of low affinity organic acid carriers. The first and rate-limiting step of long-chain FAO is CPT-I (Figure 34.2, step 1). This enzyme catalyzes the conversion of a long-chain fatty acyl-CoA to a fatty acylcarnitine. There are three isoforms, which are products of three separate genes. CPT-IA is the isoform expressed in liver, kidney, brain, fibroblasts, and leukocytes; CPT-IB is expressed in muscle and white adipose tissue; and CPT-IC is localized to brain and testes but its function in FAO is unclear. The acylcarnitine conjugates are then transported into the mitochondria by carnitine:acylcarnitine translocase (Figure 34.2, step 2). Once inside the mitochondria, the reaction is reversed by CPT-II, and free carnitine and a long-chain fatty acyl-CoA are released (Figure 34.2, step 3).
Figure 34.2 Details of the fatty acid oxidation cycle. Long-chain acyl-CoAs are imported into the mitochondrial matrix by the action of the carnitine cycle (steps 1–3), while medium-chain acyl-CoAs enter the mitochondria directly. Once in the matrix, acyl-CoAs undergo a set of four reactions (steps 5–7) generating one molecule of acetyl-CoA and an acyl-CoA substrate that is two carbons shorter. The substrate carbons from which acetyl-CoA is derived are depicted in red. Abbreviations are as in the text.
Fatty acyl-CoAs undergo β-oxidation in a repeating four-reaction cycle [1, 5]. Each cycle releases one molecule of acetyl-CoA and a chain-shortened fatty acid (Figure 34.2, steps 4–7). Each step is catalyzed by a series of carbon chain length optimum enzymes (Table 34.1), encompassing long-chain, medium-chain, or short-chain substrates. Greater than 95% of dietary fatty acids as well as the majority of endogenous lipid stores are comprised of long-chain fatty acids. Medium- and short-chain fatty acids are generated primarily from the successful chain shortening of long-chain fats and/or short-chain fatty acids from colonic fermentation. Most diets contain low concentrations of medium- or short-chain fatty acids (<5% of total lipid) unless specifically supplemented with medium-chain triglycerides (MCT).
Acyl-CoA Dehydrogenases
The first intra-mittochondrial reaction of FAO is performed by one of five substrate optimized acyl-CoA dehydrogenases (ACADs) (Figure 34.2; step 4) [10]. The dehydration reaction generates a 2-enoyl-CoA product with a double bond between the α- and the β-carbon. ACADs require an adenine dineucleotide (FAD) as a cofactor (generated from riboflavin) and produce reducing equivalents (FADH2). The ACAD is re-oxidized by electron transfer flavoprotein (ETF), which in turn is oxidized by electron transfer flavoprotein:coenzyme Q oxidoreductase (also known as ETF dehydrogenase: ETFDH), which transfers its electrons to coenzyme Q of electron transport chain complex III. Approximately a third of the energy from long-chain fatty acid is generated through this series of redox reactions, while the remainder is derived from further metabolism of acetyl-CoA by the TCA cycle.
Very-Long-Chain Acyl-CoA Dehydrogenase
Straight-chain fatty acids 12–18 carbons in length are metabolized by very-long-chain acyl-CoA dehydrogenase (VLCAD) [6]. This enzyme was originally isolated from rat liver mitochondria and differed from the other human acyl-CoA dehydrogenases because it is a homodimer bound to the inner mitochondrial membrane [11]. The ACADVL gene contains 20 exons, is about 5.4 kb long, and has been mapped to human chromosome 17p11.13-p11 [12].
Long-Chain Acyl-CoA Dehydrogenase
Long-chain branched or unsaturated fatty acids are the likely substrates for long-chain acyl-CoA dehydrogenase (LCAD), an enzyme found within the mitochondrial matrix [13]. This enzyme is a homotetramer of four identical subunits; each subunit binds one molecule of FAD. Lauric acyl-CoA (C12) is the preferred substrate [14, 15]. Expression of ACADL is very low in human liver and cardiac and skeletal muscle, and thus the enzyme likely plays more of a boutique function in cellular metabolism rather than being an important component of energy generation [16]. ACADL encodes LCAD protein and is mapped to chromosome 2q34-p35 [17]. Patients previously described with LCAD deficiency have all been subsequently found to have VLCAD deficiency [17]. To date, no deficiency disorder of LCAD has been identified in humans.
Acyl-CoA Dehydrogenase 9
Long-chain polyunsaturated fatty acids may also be oxidized by acyl-CoA dehydrogenase 9 (ACAD9). This enzyme was originally shown to have a substrate preference for polyunsaturated fatty acids and is the primary long-chain acyl-CoA dehydrogenase expressed in the CNS [18]. However, it is now recognized to also play a role as a respiratory chain complex I assembly factor, and it most frequently manifests with cardiomyopathy and skeletal myopathy [19].Traditionally, the CNS is believed to be glycolytic and to use only glucose and ketones as energy substrates. However, all of the FAO enzymes are synthesized in the CNS [20]. Two additional ACAD proteins were recently described in human and mouse CNS, ACAD10 and ACAD11, whose physiologic substrates remain unknown [21]. However, mice deficient in ACAD10 develop insulin resistant diabetes and obesity. The expression of multiple ACAD enzymes as well as the other FAO enzymes in the CNS suggests an important role in neuronal function but the role FAO plays in energy metabolism or in other essential CNS processes remains to be elucidated.
Medium-Chain Acyl-CoA Dehydrogenase
Chain-shortened acyl-CoAs (C6–12) become the substrate of medium-chain acyl-CoA dehydrogenase (MCAD). The gene encoding this enzyme, ACADM, contains 12 exons and maps to chromosome 1p31.1 [22]. The enzyme is a homotetramer located in the mitochondrial matrix, similar to LCAD (Figure 34.3). MCAD is an important enzyme in humans, and deficiency of MCAD is the most common FAO defect in Caucasian populations.
Figure 34.3 X-ray crystal structure of MCAD. The top half of the figure shows the monomer. The bottom half of the figure shows the mature homotetramer. Substrate (purple) and FAD (gold) are modeled into the monomer.
Short-chain Acyl-CoA Dehydrogenase
Short-chain acyl-CoA dehydrogenase (SCAD) catalyzes the dehydrogenation of short-chain acyl-CoAs (C4–6). The gene encoding it, ACADS, consists of 10 exons spanning 13 kb of DNA on chromosome 12q [23]. SCAD is also a homotetramer located in the mitochondrial matrix like LCAD and MCAD, suggesting a common ancestral origin.
2-Enoyl-CoA Hydratases
The second step in FAO is hydration of the 2-enoyl-CoA to form a 3-hydroxyacyl-CoA (Figure 34.2, step 5). There are two separate hydratase enzymes. Long-chain substrates are acted upon by long-chain enoyl-Co hydratase, a component of the mitochondrial trifunctional protein (TFP) [24, 25]. TFP also catalyzes two additional steps of FAO (see “Trifunctional Protein” below). Medium- and short-chain fatty acids are the substrate for the enzyme short-chain 2,3-enoyl-CoA hydratase (also known as crotonase).
Trifunctional Protein
The second through fourth reactions in long-chain FAO are catalyzed by TFP, which is bound to the inner mitochondrial membrane. Its activities include long-chain enoyl-CoA hydratase, long-chain 3-hydroxyacyl-CoA dehydrogenase, and long-chain ketoacyl-CoA thiolase activities [24, 25]. Mitochondrial TFP is a heterotetramer composed of 2α- and 2β-subunits encoded by two different genes, HADHA and HADHB (Figure 34.4). Both genes are located on chromosome 2p23 in a head-to-head configuration with coordinately regulated expression [26]. The long-chain acyl-CoA hydratase and the long-chain hydroxyacyl-CoA dehydrogenase activity are contained in the α-subunit and the long-chain acyl-CoA ketoacyl-CoA thialase activity is on the β-subunit.
Short-Chain Enoyl-CoA Hydratase
2,3-Enoyl-CoA hydratase is a homohexamer localized in the mitochondrial matrix and encoded by ECHS1. The gene is located on chromosome 10q26.2-q26.3 and is highly expressed in human liver, as well as in fibroblasts and muscle [27]. 2,3-Enoyl-CoA hydratase acts on fatty acids of C4 or longer but activity toward long-chain fatty acids decreases with increasing chain length.
3-Hydroxyacyl-CoA Dehydrogenases
3-Hydroxyacyl-CoAs are substrates for the 3-hydroxyacyl-CoA dehydrogenases, the third step of FAO. The hydroxyl group is dehydrogenated to a keto group at carbon 3 (Figure 34.2, step 6). There are two 3-hydroxyacyl-CoA dehydrogenases: LCHAD, which is part of the TFP (see “Trifunctional Protein” above), and medium-/short-chain 3-hydroxyacyl-CoA dehydrogenase, encoded by HADH. Niacin adenine dinucleotide (NAD+) is a required cofactor for both enzymes. Reducing equivalents (NADH) generated in this step are channeled to complex I of the electron transport chain. The medium-/short-chain 3-hydroxyacyl-CoA dehydrogenase is a soluble mitochondrial matrix protein with broad activity toward C4–C16 fatty acids. However, its highest activity is toward 3-hydroxydeconyl-CoA (C10) [28]. Historically, the enzyme was designated as short-chain 3-hydroxydeconyl-CoA dehydrogenase, but this was misleading as there is a 3-hydroxydeconyl-CoA dehydrogenase in the brain that plays a role in brain development [29]. The protein involved in FAO is most correctly referred to as 3-hydroxyacyl-CoA dehydrogenase or HADH but is still often called SCHAD in many publications.
3-Ketoacyl-CoA Thiolases
The final cleavage step is catalyzed by three distinct thiolase enzymes: the long-chain 3-ketoacyl-CoA thiolase component of TFP (see “Trifunctional Protein” above) for long-chain substrates; medium-chain 3-ketoacyl-CoA thiolase for medium-chain substrates; and short-chain 3-ketoacyl-CoA thiolase (β-ketothiolase) for short-chain substrates (Figure 34.2, step 7) [1]. The product of this reaction is one molecule of acetyl-CoA and a chain-shortened fatty acyl-CoA.
Medium-Chain Ketoacyl-CoA Thiolase
The medium-chain ketoacyl-CoA thiolase has also been called mitochondrial 3-oxoacyl-CoA thiolase. The mRNA for this thiolase is highly expressed in human muscle, liver, and fibroblasts. There is some substrate overlap between medium-chain and short-chain 3-ketoacyl-CoA thiolases. The former can cleave C4 into 2 acetyl-CoA molecules as well as cleave longer fatty ketoacyl-CoA moieties, with highest activity toward C10. It is believed to be the primary thiolase in the medium- and short-chain FAO pathway.
Short-Chain 3-ketoacyl-CoA Thiolase
The short-chain 3-ketoacyl-CoA thiolase is encoded by ACAT1 on chromosome 11q22.3-q.23.1, which contains 12 exons and 11 introns. The cDNA is 1.5 kb and encodes a 427 amino acid residue peptide; the enzyme is a homotetramer of four identical 41 kDa subunits. It cleaves four carbon substrates into two molecules of acetyl-CoA and is thought to be primarily involved in ketone body synthesis.
Oxidation of Odd-Chain Fatty Acids
Very small amounts of odd-chain fatty acid are found in dairy food products, are digested and absorbed into circulation, and must be stored or oxidized [22]. Odd-chain fatty acids are also generated from catabolism of complex lipids such as cholesterol. These unique fatty acids are oxidized in the same four-step process as described above to yield multiple acetyl-CoAs and one C3 intermediate, propionyl-CoA. Propionyl-CoA is converted to succinyl-CoA, a tricarboxylic acid (TCA) cycle intermediate by three additional enzymes (Figure 34.4). First, propionyl-CoA is carboxylated to D-methylmalonyl-CoA by propionyl-CoA carboxylase, a biotin-dependent enzyme. D-Methylmalonyl-CoA is then converted to its L isomer, L-methylmalonyl-CoA, by methylmalonyl-CoA epimerase. L-Methylmalonyl-CoA is converted to succinyl-CoA by methylmalonyl-CoA mutase, a vitamin B12-dependent enzyme. The addition of succinyl-CoA to the pool of TCA cycle intermediates via the oxidation of odd-chain fatty acids or branched-chained amino acids is a process termed anaplerosis [30, 31].
Unsaturated Fatty Acids
Unsaturated fatty acids such as oleic acid (18:1 n-9), linoleic (18:2 n-6), and linolenic (C18:3 n-3) constitute a significant portion (6–8%) of the total energy consumed by humans. Polyunsaturated fatty acids can be used for membrane synthesis and complex lipid structures or oxidized to produce ATP. These fatty acids undergo β-oxidation as for saturated substrates until the double bond is reached [32, 33]. The double bonds typically occur every third carbon in a cis formation. A double bond between the C3 and C4 atoms (cis-3-enoyl- CoA) is rearranged to a trans double bond by enoyl-CoA isomerase. The trans-2-enoyl-CoA becomes the substrate for long-chain acyl-CoA hydratase or short-chain enoyl-CoA hydratase depending on the fatty acid chain length and continues through the beta-oxidation cycle. When the double bond is located between the C2 and C3 atoms, 2,4-dienoyl-CoA reductase changes the compound into a trans-3-enoyl-CoA. Enoyl-CoA isomerase rearranges the bond to form 2-enoyl-CoA, which proceeds through the normal β-oxidation cycle.
Final Products of Fatty Acid Oxidation
The product for each cycle of the FAO system is a chain-shortened fatty acyl-CoA and one molecule of acetyl-CoA. In liver and muscle, acetyl-CoA is a substrate for the TCA cycle, which generates additional reducing equivalents for OXPHOS production of ATP, CO2, and water. Energy generated through FAO spares glycogen and prevents glucose depletion. Acetyl- CoA generated from FAO is also necessary for the liver to make ketone bodies during periods of fasting. Ketone bodies are released into circulation and provide an alternative fuel for the brain.
Fatty Acid Oxidation Disorders
Carnitine Shuttle Defects
Defects in the carnitine-dependent transport of long-chain fatty acids into the mitochondria include carnitine transport defects (organic cation transporter 2; OCTN2), CPT-IA deficiency, CPT-II deficiency, and carnitine/acylcarnitine translocase deficiency [34].
Carnitine Transport Defects
Carnitine is consumed in the diet from meat, fish, and dairy products but it can also be synthesized from lysine and methionine in the liver. A primary defect in carnitine synthesis has been proposed to be a risk factor in autism in a single study but no follow-up studies have been published, and no other systemic synthetic defects have been reported [35]. Primary/systemic carnitine deficiency more typically refers to a defect in tissue OCTN2 [34]. Tissues such as muscle and heart cannot synthesize carnitine and must take up circulating carnitine via the transporter. Defects in OCTN2 lead to carnitine depletion in muscle, liver, and heart. Muscle depletion of carnitine leads to weakness; cardiac depletion causes cardiomyopathy and arrythmias; and hepatocellular depletion can cause asymptomatic hepatic steatosis or fulminant Reye-like syndrome with hepatic failure, any of which can be precipitated during stress or minor periods of starvation. Sudden unexpected death in infancy or older individuals has been reported in patients with OCTN2 defects. A common variant is present in Faroe Islands and may remain asymptomatic until adulthood.
Carnitine Palmitoyltransferase-IA
Deficiency of CPT-IA causes fasting hypoketotic hypoglycemia and occasionally hepatocellular damage. The heart and muscle are rarely involved because CPT-IA is the liver isoform of the enzyme. One case of CPT-IA deficiency presented with muscle pain and rhabdomyolysis in a previously asymptomatic adult [36]. Hepatic presentation of CPT-I deficiency in one sibling and muscle presentation in another sibling in a family has also been reported. In CPT-IA deficiency, total and free plasma carnitine are characteristically elevated; this observation is unique among all known FAO defects and is otherwise seen only in association with carnitine supplementation or renal insufficiency.
Carnitine Palmitoyltransferase-II
An infantile form of complete CPT-II deficiency presents with severe hypoglycemia, myopathy, and cardiomyopathy and often results in death [37]. Plasma free carnitine is low with elevated acylcarnitines. A milder form is caused by a common mutation that leads to residual enzyme activity and presents with lipid myopathy, recurrent rhabdomyolysis, and myoglobinuria in young adults. The combination of one severe mutation and one mild leads to onset of disease in later infancy or early childhood. Carnitine/acylcarnitine translocase deficiency is clinically similar to infantile CPT-II deficiency. Patients often present in the neonatal or infancy period with severe hypoketotic hypoglycemia; hyperammonemia, probably from liver failure, and elevated acylcarnitines associated with intercurrent illness [26]. Cardiac arrhythmias are common.
Long-Chain Fatty Acid Oxidation Disorders
Very-Long-Chain Acyl-CoA-Dehydrogenase Deficiency
Deficiency of VLCAD presents with a spectrum of symptoms ranging from a severe infantile presentation with hypoglycemia, hyperammonemia, and liver steatosis and dysfunction similar to MCAD deficiency, to recurrent rhabdomyolysis in adolescents and young adults mimicking CPTII deficiency [38, 39]. Hypoglycemia is rare in late onset patients. Early onset patients typically transition to the muscle phenotype later in childhood (typically age four to six years). Cardiomyopathy may develop at any age and may be dilated or hypertrophic [38, 40]. Cardiac arrhythmias have been reported. Cardiomyopathy may resolve with the initiation of treatment with carbohydrates and MCT supplements but may also progress to demise. VLCAD deficiency is now diagnosed by newborn screening prior to the onset of symptoms in the USA and many other countries [39, 41].
During acute illness, characteristic plasma long-chain acylcarnitines are increased including C14:0, C14:1, C16:0, C16:1, C18:0, C18:1 and C18:2 acylcarnitine esters [42]. Rhabdomyolysis and myoglobinuria are often related to prolonged exercise, an illness with fever or poor oral intake, cold temperatures, or a combination of these factors. Creatine phosphokinase can be extremely elevated and myoglobinuria can cause renal failure requiring hemodialysis.
Adults most often experience exercise intolerance and myoglobinuria without hypoglycemia, but may also have muscle pain and weakness without frank rhabdomyolysis. Symptoms may begin in adolescence and continue intermittently for decades prior to a diagnosis of VLCAD deficiency. Patients may also present with an acute rhabdomyolysis event and no prior history of muscle disease [38–40]. More than 100 different mutations spanning the ACADVL gene have been reported and no common mutation has been identified [43, 44].
Acyl-CoA Dehydrogenase 9
Deficiency of ACAD9 was originally described in three patients with a clinical presentation similar to other FAO disorders including hypoketotic hypoglycemia, fulminant liver failure, and dilated cardiomyopathy [45]. In addition, neurological symptoms including cerebral stroke were present.
Since the initial report, ACAD9 has been shown to be an essential protein for mitochondrial complex 1 assembly and that this function is separate from its enzymatic one [46]. Mutations in ACAD9 are now recognized as a common cause of mitochondrial disease ETC complex 1 deficiency [19]. Two major phenotypes are seen; predominantly cardiomyopathy with milder muscular symptoms, and a more systemic disease as seen in the original patients. The former phenotype is associated with mutations that preserve the ACAD enzymatic functions while the latter are caused by mutations that affect both functions [46]. Many of the isolated respiratory chain deficient patients respond to riboflavin supplementation [19].
Long-Chain 3-Hydroxyacyl-CoA Dehydrogenase
Mutations in the HADHA and HADHB genes can lead to a relative selective loss of the LCHAD activity of TFP or loss of all three TFP activities [47, 48]. All patients share some aspects of clinical course with other fatty acid oxidation disorders (FAODs) including hypoketotic hypoglycemia associated with metabolic acidosis, hepatocellular dysfunction, and sometimes cardiomyopathy. Rhabdomyolysis is common in patients beyond the first few years of life. However, pigmentary retinopathy with vision loss during childhood and progressive peripheral neuropathy are complications specific to LCHAD/TFP deficiency [49]. The former tends to be more severe in isolated LCHAD deficiency, while the latter is usually worse in complete TFP deficiency. While the underlying etiology of the hypoketotic hypoglycemia appears to be a deficiency of energy from depleted carbohydrate stores, the etiology of the other complications is not completely understood [50]. Elevated plasma long-chain hydroxyacylcarnitines, including C16:0-OH, C16:1-OH, C18:0-OH, C18:1-OH, C18:2-OH, are diagnostic of LCHAD or TFP deficiency.
Isolated LCHAD deficiency results from the selective loss of LCHAD activity and relative preservation of hydratase and 3-ketoacyl-CoA thiolase activity of TFP. A common missense mutation, (c.G>C1528) accounts for 87% of the alleles in LCHAD deficiency, affecting the catalytic residue in the active site for this activity [26, 51]. This common mutation decreases LCHAD activity but minimally alters the level of protein of both mitochondrial TFP subunits. Other mutations in both the α- and β-subunits have been shown to result in the loss of all three enzyme activities and TFP deficiency.
There appear to be some clinical differences between LCHAD and TFP deficiencies. Patients who are homozygous for the common LCHAD mutation are more likely to develop progressive chorioretinopathy leading to vision impairment than patients who have β-subunit mutations [50]. The infantile form of TFP deficiency appears to be severe, often resulting in neonatal death [47, 48]. Milder forms of TFP deficiency are associated with severe peripheral neuropathy, impairing mobility, more often than isolated LCHAD deficiency. Patients who are heterozygotes for the common LCHAD mutation and a private mutation may develop some aspects of both the chorioretinopathy with vision loss and peripheral neuropathy with impaired mobility.
Medium-Chain Acyl-CoA Dehydrogenase Deficiency
Deficiency of MCAD is the most common defect in FAO, with an incidence of approximately one in 9,000 live births in western European populations [52]. It is less common in Asians and Africans and the overall incidence in the USA is ~1:26,000 births. The typical acute clinical presentation includes fasting or illness induced hypoketotic hypoglycemia, often associated with metabolic acidosis and hepatocellular dysfunction [53]. Hyperammonemia may be present. Micro- and/or macrovascular steatosis is often observed in symptomatic patients, but typically resolves when patients are well. Prior to the advent of newborn screening for this disorder, as many as half of all patients presented with sudden unexplained death [54]. Even with newborn screening, sudden unexpected death may occur in the first few days after birth (almost exclusively in breast-fed infants) prior to the report of the newborn screen results [55]. Patients become less prone to episodes of decompensation in early childhood (typically by age six to seven years), and symptoms in adults are rare but may be induced by prolonged fasting or severe intercurrent illness. Asymptomatic adults have been described.
In 1990, a common mutation in ACADM, a c.985A>G point mutation, was identified resulting in a lysine to glutamic acid substitution at position 304 of the mature protein [56]. This missense mutation was subsequently shown to account for a large majority of the alleles in identified patients, although with newborn screening identifying affected individuals, the prevalence of this mutation has changed somewhat [52]. Other point mutations have been identified in the first 11 of 12 exons but the c.985A>G point mutation still accounts for the majority of disease-causing alleles [57]. The c.985A>G mutation leads to the normal transcription and translation of a mutant protein that is appropriately imported into mitochondria, but then fails to fold properly to a mature monomer [58].
In countries with newborn screening for MCAD deficiency, most affected infants remain asymptomatic and have an excellent outcome [53]. The vast majority of affected patients who are treated are completely healthy, although they may need to receive intravenous fluids containing glucose when ill or fasting to prevent metabolic crisis. Some neurological impairment has been noted later in life in MCAD-deficient patients, particularly in unscreened populations, but the evidence for neurological impairment in treated patients who have avoided metabolic crisis is scant. Deficits in learning and speech/language communication have been reported in symptomatic individuals prior to newborn screening but not in screened and early effectively treated populations [59].
Short-Chain Acyl-CoA Dehydrogenase Deficiency
Deficiency of SCAD was originally described in patients with a broad spectrum of symptoms including failure to thrive, hypoglycemia, hypotonia, developmental delay, and seizures, but based on patients identified through newborn screening, it is now generally believed to be a biochemical of no clinical relevance [60]. In fact, it is likely that most patients described in the literature with SCAD deficiency had ethylmalonic aciduria due to the presence of one of two common sequence variants in the ACADS gene (c.511>CT and c.625 C>A) [61]. These variants slightly affect enzyme activity leading to increased production of ethylmalonic acid, but are of no clinical consequence [62]. As these variants are present in up to 14% of the general population the associated ethylmalonic aciduria can be coincidentally identified in any clinical scenario.
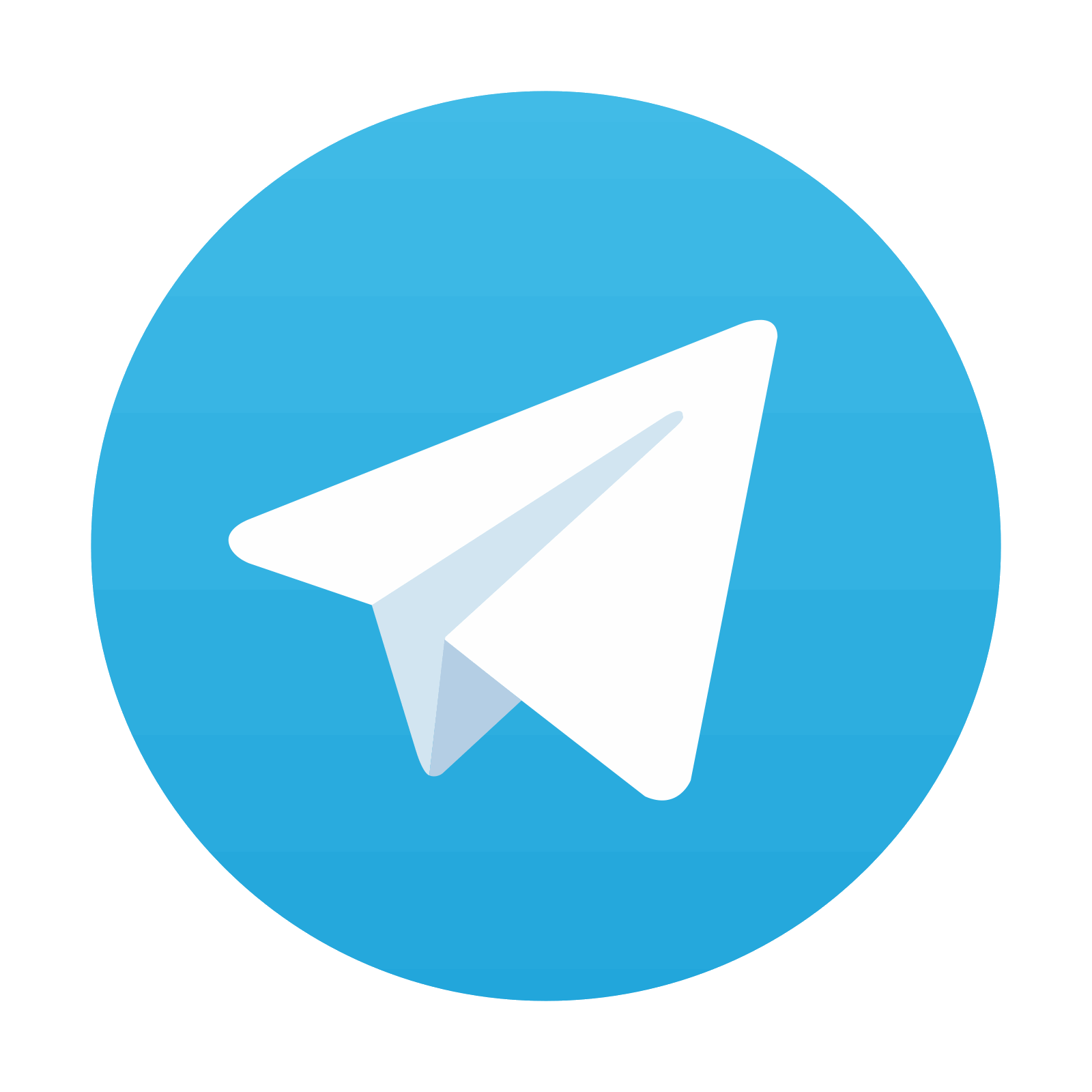
Stay updated, free articles. Join our Telegram channel
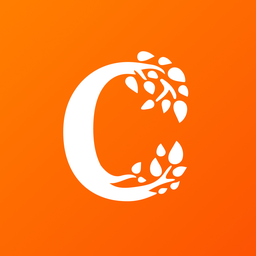
Full access? Get Clinical Tree
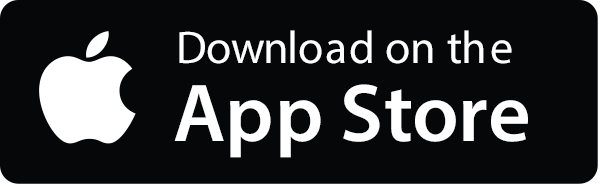
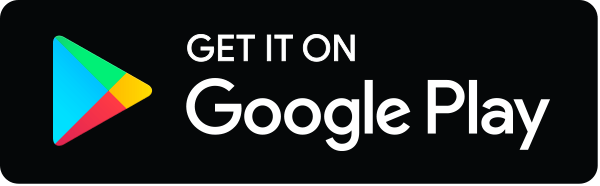
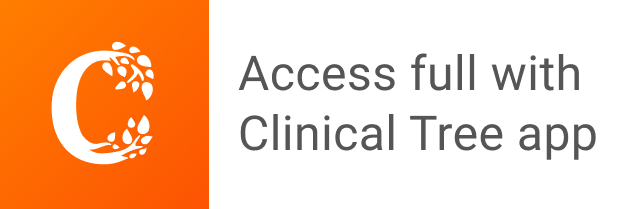