Abstract
Bile formation and flow is an essential physiological function. Adequate bile flow is necessary for innumerable daily functions including digestion, metabolism, growth, development, toxin elimination and adaptation to liver disease. Cholestasis, a slowing or, in its extreme form, cessation of bile flow, is a pathophysiological process that interrupts the necessary functions of bile while biliary constituents accumulate intrahepatically [1–3]. This accretion of bile leads to liver damage from the multiple effects of retained biliary constituents, including various lipids, toxins, and as a principal effector – bile acids [4–6]. These effects of cholestasis, regardless of cause, are amplified and accelerated in the infant, rendering the consequences of unaddressed neonatal cholestasis urgent [1, 7–9]. A molecular understanding of the determinants of bile formation is helpful to link these individual solutes to the responsible genes and functions in normal biology and in cholestasis. Bile formation and flow relies upon membrane transporters of biliary solutes, and their regulators, that function to deliver each of the molecular constituents into bile. The focus of this chapter is to lay the groundwork of current knowledge of the mechanisms of bile formation and to explore normal and pathophysiological processes when these mechanisms are impaired. Moreover, very recent work based upon the knowledge of bile formation has led to new therapeutic targeting, with the goal of filling the serious gap in effective anti-cholestatic therapies.
Introduction
Bile formation and flow is an essential physiological function. Adequate bile flow is necessary for innumerable daily functions including digestion, metabolism, growth, development, toxin elimination and adaptation to liver disease. Cholestasis, a slowing or, in its extreme form, cessation of bile flow, is a pathophysiological process that interrupts the necessary functions of bile while biliary constituents accumulate intrahepatically [1–3]. This accretion of bile leads to liver damage from the multiple effects of retained biliary constituents, including various lipids, toxins, and as a principal effector – bile acids [4–6]. These effects of cholestasis, regardless of cause, are amplified and accelerated in the infant, rendering the consequences of unaddressed neonatal cholestasis urgent [1, 7–9]. A molecular understanding of the determinants of bile formation is helpful to link these individual solutes to the responsible genes and functions in normal biology and in cholestasis. Bile formation and flow relies upon membrane transporters of biliary solutes, and their regulators, that function to deliver each of the molecular constituents into bile. The focus of this chapter is to lay the groundwork of current knowledge of the mechanisms of bile formation and to explore normal and pathophysiological processes when these mechanisms are impaired. Moreover, very recent work based upon the knowledge of bile formation has led to new therapeutic targeting, with the goal of filling the serious gap in effective anti-cholestatic therapies.
The processes of bile formation, causes of cholestasis, and the liver’s adaptation to cholestasis are dynamic, inter-related and engage features of liver development, synthesis, transport and molecular signaling. A focus of adaptation to cholestasis hinges upon how the principal solute, bile acids, are both metabolic signaling molecules as well as mediators of toxicities [10]. In this chapter, attention will focus upon the basic mechanisms of bile formation, as well as the genetic and acquired pathways that lead to cholestasis.
Basic Physiological Principles of Bile Formation
Bile is an aqueous solution composed principally of bile acids, phospholipids, cholesterol, electrolytes, modified and unmodified metabolites and toxins. There are generally two broad categories that outline the need for adequate bile flow: enhancing nutrition and excretion of toxins. The hepatocellular synthesis and canalicular secretion of bile acids, along with their enterohepatic circulation (see “Enterohepatic Circulation” below) are the prime drivers of bile flow. Bile acids from the diet are minimal. In addition to bile acid secretion, the other main contributors to bile flow are from water, glutathione and bicarbonate-rich electrolytes derived from cholangiocytes lining the biliary tract [11]. From a nutritional standpoint, the delivery of bile acids to the intestinal lumen allows for solubilization and efficient absorption of fats and fat-soluble vitamins [12]. This is important at all ages, but is most crucial during infancy, as this is the time of greatest growth dependent upon adequate delivery and absorption of essential nutrients. As an excretory route, bile is the preferred means for metabolic end-products (endobiotics) and ingested toxins (drugs and xenobiotics) to be delivered to the intestine, destined for fecal elimination. These dual intertwined functions of bile flow for proper growth and detoxification are hampered due to a variety of liver diseases [13]. Moreover, the hepatic consequences of cholestatic disorders, more rapid and profound in infancy, are the major pathophysiological etiology of serious liver diseases in infants and children [9].
The Enterohepatic Circulation of Bile Acids
Several excellent reviews provide detailed explanations of the molecular and cellular components of the enterohepatic circulation of bile acids [6, 10, 12, 14]. In brief, bile acids are synthesized solely within hepatocytes from cholesterol precursor molecules, whose end-products after a multi-step enzyme process are the primary bile acids cholic acid and chenodeoxycholic acid (see Chapter 33) [15]. These two bile acids are conjugated to glycine (and to a lesser extent, to taurine) – conjugated bile acids are the substrate for the sole canalicular transporter of bile acids, the bile salt export pump (BSEP; encoded by ABCB11) [16]. After secretion into the canalicular space, conjugated bile acids travel down the biliary tree to be stored in the gall bladder awaiting hormonal stimulation for delivery into the duodenum after a meal. All components of bile follow this same route led by bile acids as the principal biliary solute (upwards of 30 mM concentrations in gallbladder). There are minimal alterations of conjugated bile acids in the intestine, until their influx into the distal ileum. The enterohepatic circulation of bile acids has been known since the mid-1800s, but it was not until 1963 when Borgstrom and Hofmann clearly identified the distal ileum as the site for > 95% reclamation of luminal bile acids [17]. In 1994, Paul Dawson’s lab identified and cloned the gene that encodes the ileal bile acid transporter (IBAT, aka apical sodium-dependent bile acid transporter, ASBT, SLC10A2) [18]. Similar to canalicular BSEP in the hepatocyte for secretion of bile acids, ASBT is the sole means effecting conjugated bile acid transport in the ileum.
After uptake and transport across the enterocyte apical membrane, conjugated bile acids are secreted into the portal circulation by a heterodimer (Ost-α/Ost-β; encoded by SLC51A/SLC51B) [19], where they travel back to the hepatocyte, with uptake from the portal circulation primarily via the Na-dependent taurocholate cotransporting polypeptide NTCP (SLC10A1) [20]. After re-importation of cross the basolateral membrane, conjugated bile acids, they are ready for rapid hepatocellular transport to the apical surface to be re-excreted by BSEP into the canalicular space. This re-circulation mechanism is highly efficient: >90% of bile acids are maintained in this loop throughout a 24-hour cycle, with each bile acid cycling upwards of four to six times. Recent studies utilizing molecular tracers of bile acid flux (11C-cholylsarcosine) in humans has provided detailed concentrations, transit and tissue dwell times that were previously unknown [21]. In the normal adult, bile flows at ~ 0.26 ml/min, and the average bile acid dwell time after uptake from the portal circulation in the liver was only 2.5 minutes before secretion into bile. There is a marked uphill concentration gradient from portal blood→liver→bile of 1:2.6:1,400 respectively, attesting to the need for active bile acid transport mechanisms. Some bile acids escape ileal uptake, and after exposure to certain bile acid modifying bacteria in the colon, develop an array of secondary bile acids that are more hydrophobic than the primary bile acids [22]. These gut-modified bile acids, mainly deoxycholic and lithocholic acids, are transported to the liver, where upon import, become part of the bile acid pool after conjugation. Taken together, there are genetic, developmental, dietary, pharmacological and microbial contributions to the actual bile acid composition in the pool.
Molecular Determinants of Bile Formation and Flow in the Liver
The main driving force for bile flow is the hepatic synthesis, canalicular secretion and portal uptake that forms the centerpiece of the recirculation of bile acids [5]. In humans, conjugated bile acids are efficiently taken up from the portal circulation primarily via the NTCP. Interestingly, NTCP was recently identified as the major site of entry into hepatocytes of the hepatitis B virus (HBV) [23], and variants in SLC10A1 can alter HBV infectivity and replicative efficiencies [24–26]. Moreover, a potent inhibitor of NTCP, myrcludex, can not only interfere with entry of HBV into hepatocytes, but can markedly impair the entry of conjugated bile acids from the portal circulation, leading to a first-ever pharmacological means to inhibit re-entry of bile acids into the hepatocyte[27, 28]. Recent reports have identified severe functional variants in SLC10A1 as a cause of hypercholanemia (elevated serum bile acids) [29, 30]. Individuals with functional variants of SLC10A1 do not appear to have significant liver disease or pruritus. The genetics of variants in SLC10A1 are beginning to help explain some inter-individual differences in serum bile acid uptake and recirculation.
Bile acids are rapidly transported across the cytoplasm of hepatocytes mainly via unknown mechanisms, and efficiently secreted into the canalicular lumen via an ATP cassette transporter, BSEP [31, 32]. The secretion of bile acids against the steep concentration gradient is the ultimate, rate-limiting step of bile secretion (Figure 3.1). As expected, when ABCB11 is mutated, bile acid flow is reduced and bile acids are retained within hepatocytes, leading to the liver disease known as progressive familial intrahepatic cholestasis (PFIC) type 2 (see Chapter 13). The other main solutes in bile, phospholipids, cholesterol, and bilirubin conjugates, are also secreted into bile via substrate-specific ABC transporters. Phospholipids are secreted via a “floppase,” the multidrug resistance-associated protein 3 (MDR3; encoded by ABCB4), which, when mutated, leads to the disease PFIC3 [33, 34].
Figure 3.1 Roles for critical hepatic transporters in the formation of bile and adaptation to cholestasis.
The sinusoidal surface is shown on the left and the canalicular surface on the right. Diseases associated with defects in specific genes for canalicular transporters are given on the right. Note that bile acids have several means of transport across the sinusoidal membrane (although NTCP is the principal means in humans), while there is one canalicular bile acid transporter, the bile salt export pump (BSEP). These transporters allow for fine tuning of intracellular bile acid concentrations as a means to adapt to a variety of cholestatic conditions. The dotted line shows the main path for bile acid flux across the hepatocyte. NTCP, Na+/taurocholate cotransporting polypeptide; OATP, organic acid-transporting polypeptide; OST, organic solute transporter; MRP, multidrug resistance-associated protein; FIC1, familial intrahepatic cholestasis 1; MDR, multidrug-resistance protein. Official gene designations are noted in the filled black boxes. * indicates genes that have been associated with cholestasis when mutated, while ** indicates therapeutic targets.
Cholesterol is secreted via two half-transporters combined of the ABC family (encoded by ABCG5 and ABCG8, respectively); if either gene is mutated, this leads to the disease sitosterolemia, and generally does not lead to liver disease. Finally, conjugated bilirubin is excreted into bile primarily via the multidrug resistance-associated protein 2 (MRP2, encoded by ABCC2); mutation of this gene leads to Dubin–Johnson syndrome [35]. Detailed exome sequencing of cholestatic patients has recently indicated that biallelic mutations in ABCC2 may lead to neonatal cholestasis – i.e., patients who not only have elevated serum direct bilirubin levels, but also elevated serum bile acid levels [36]. It is quite possible that distinctions between conjugated bilirubin transport and conjugated serum bile acid recirculation may be inter-linked especially in the newborn period, and not just separate determinants of canalicular transport.
Less well-defined is the role that cholangiocytes play in modifying and contributing to bile composition. In addition to chloride secretion via CFTR, bicarbonate secretion across the apical membrane provides protection to the lumen of the biliary tree via a “bicarbonate umbrella” [37–41]. ASBT is expressed on the apical surface of cholangiocytes, although roles in modulating bile flow and contributing to intracellular signaling remain to be determined. Finally, other cholangiocyte-derived secretions across the apical membrane and molecular sensors of biliary constituents through the sole primary cilium contribute to the flow and composition of bile [42–44].
General Principles of the Pathogenesis of Cholestasis and Adaptation
The definition of cholestasis is impairment of bile flow, commensurate with intrahepatic retention of bile. This can be complete as in common bile duct obstructions (e.g., biliary atresia, choledochal cyst, choledocholithiasis); or slowed as in several defined genetic conditions (e.g., ABCB11 deficiency) or due to pruning of the biliary tree in the developmental cholangiopathy Alagille Syndrome (ALGS, see Chapter 14). The constant need for maintenance of bile flow leads to significant derangements consequent to biliary retention. A broad knowledge of the normal concentrations of bile acids in various tissues in health, and the manifold means of the liver to provide bile acid homeostasis will help understand the dynamic processes engaged in the cholestatic liver.
Total bile acid concentrations in peripheral circulation are generally <10 μmol/L, while those in portal blood varies from below 10–20 μmol/L between meals to upwards of 100 μmol/L postprandially, while the significant concentrations in bile are upwards of 20–100 mmol/L [6, 21].Therefore, the highest concentration of bile acids is in the canalicular and biliary lumens, and it is the intracellular retention of bile acids that appears to be the most important disease-producing consequence of cholestasis, and is the focus of adaptation. Recent data indicate that in obstructive cholestasis there is a breach of the apical surface of the biliary tree allowing for retrograde seepage of the high concentration of bile acids back into hepatocytes [13, 45]. This important recent finding indicates that there isn’t limitless protection afforded by the hepatocyte to high concentrations (and perhaps pressure) of canalicular bile, which at a certain threshold leads to increasing concentrations of bile acids retained within hepatocytes.
Recruitment of sinusoidal transporters by bile acid-activated gene expression helps to unload the hepatocyte somewhat, but the processes are not complete. This leads to prolonged retention of bile acids within the liver, secretion of various damage signals to recruit activated Kupffer cells, stellate cells, and myofibroblasts, with consequent increased production of cytokines and progression of fibrosis. A counter-productive response to increasing intracellular concentrations of bile acids is a maintenance, or even increase, in the expression of the BSEP gene, due to positive transcriptional feedback at its promoter (Figure 3.1). Therefore, the overall effects of cholestasis, perhaps even long term, can in a broad way be ascribed to the ill effects of retained bile acids within and surrounding hepatocytes. Finally, little is known about the actual molecular causes of the enhanced susceptibility of the infant liver to cholestatic insults, although an immaturity of bile acid flux, imperfect hepatocellular and cholangiocytic adaptations, and recruitment of inflammatory cells contribute [46–49].
Over the past few years, much has been added to our understanding about how the hepatocyte responds to, and adapts to, retained bile acids. Less is known about cholangiocytes. The hepatocyte is poised to respond to retained bile acids with a coordinated approach that treats retained bile acids as if they were a damaging, foreign compound: i.e., high levels of retained bile acids as sensors and xenotoxic endobiotics. Multiple processes, both transcriptional (mainly nuclear receptor mediated) and post-transcriptional, are engaged in the hepatocyte [10, 50–52] with the overall arc to reduce the intracellular concentration by suppressing bile acid import and synthesis, reduce toxicity by sulfation and conjugation, and to increase export by sinusoidal and canalicular efflux (when possible). At the transcriptional level, bile acids are activators of at least three members of the NR superfamily – the constitutive androstane receptor (CAR), the pregnane X receptor (PXR), and the farnesoid X receptor (FXR) – and these three gene regulators are the primary means for effecting the transcriptional reprogramming of the hepatocyte in cholestasis, with FXR as the centerpiece of regulatory control in cholestasis [10, 53, 54]. Genetically modified mice with mutations in any of these genes are essentially normal, except when exposed to cholestatic conditions. In cholestasis, mice without any one or more of these three regulators rapidly develop hepatocyte apoptosis and necrosis, principally mediated by an inability to adapt to retained bile acids. Another cell membrane receptor, TGR5, has been alluded to as having a crucial role in mediating the systemic actions of bile acids, however, TGR5 is expressed on cholangiocyte and not hepatocyte membranes [55]. Importantly, in genetically normal mice, these NR-regulated adaptive mechanisms can be enhanced with small molecules that bind to the NR, lending an exciting prospect for future treatment paradigms (see below). In fact, the first FXR activator indicated for clinical use, obeticholic acid, was FDA-approved in 2016 for primary biliary cholangitis [56]. Finally, Gomez-Ospina and colleagues identified the first cholestatic patient with a pathogenic FXR (NR1H4) mutation, lending further credence to the indispensable relevance of FXR in the human neonate [57, 58]. Although the expression of some NRs has been studied in humans and experimental animals with various forms of cholestasis, there is little information on how assembly of coregulators and histone-modifying enzymes that regulate the function of NRs are involved in perpetuating and adapting to cholestasis. There are a number of possible contributory roles: (1) the production of coactivators and histone-modifying enzymes may be impaired or induced in cholestasis; (2) their recruitment to the nucleosome may be disrupted by alterations in chromatin structure; and (3) there may be active recruitment of corepressors and histone deacetylases to the promoters of critical genes. Together there are transcriptional and post-transcriptional intersecting responses to bile acid overload in cholestasis, some of which may be amenable to therapeutic targeting.
The Genetic Underpinnings of Cholestasis
There are multiple genetic mechanisms that lead to cholestasis, most involving variants in genes critical for adequate hepatobiliary transporter function or genetic variants that contribute to abnormal development or structure of bile ducts [59]. An impaired ability to transport essential biliary substances across the canalicular membrane of the hepatocyte leads to obligate retention of that substance within hepatocytes (e.g., conjugated bile acids in ABCB11 deficiency, PFIC2) while a deficiency in the ability to transport phospholipids into the canalicular lumen (ABCB4 deficiency, PFIC3), leads to bile acid-induced damage in hepatocytes and cholangiocytes [60]. Recent application of next generation sequencing (NGS) to patients with cholestasis has led to several new gene-disease associations, including those associated with neonatal sclerosing cholangitis (DCDC2) [61], familial cholestatic diseases (KIF12 and others) [62, 63], cholestasis with diarrhea (SLC51B, a component of the sinusoidal bile acid export heterodimer Ost-α/Ost-β) [64] and the biliary atresia splenic malformation syndrome (PKD1L1) [65]. It is anticipated that more genetic contributions to cholestasis will be identified over the next few years with the ready availability and utilization of NGS technologies like whole exome sequencing, especially in those patients with cholestasis in the newborn period [66, 67]. More detailed information about the specific genetic disorders that lead to various forms of cholestasis are presented in Chapters 11, 13, 14, 33 and 41.
Acquired Mechanisms of Cholestasis
In addition to the single gene defects noted above that can lead to cholestasis, it is generally more prevalent that multifactorial, or structural, mechanisms are the main participants. Of these, drug-induced, total parenteral nutrition (TPN) or sepsis/ inflammation induced mechanisms are now being recognized to have molecular underpinnings.
Sepsis-Associated Cholestasis
Osler was among the first to describe the association of non-hepatitic infections leading to a functional impairment in bile flow; “toxemic jaundice” [68]. It has been well known, but poorly understood, that such cholestasis does not result from damage or destruction of hepatocytes but is a functional impairment from either bacterial products (e.g., endotoxin), or inflammation-induced cytokines [69]. Infants in particular are more susceptible to the effects of sepsis on bile flow, perhaps due to immaturity of bile formation or adaptive mechanisms [70]. Sepsis-associated cholestasis is a principal cause of cholestasis in adults as well, although it is not usually at the top of the list of differential diagnoses. Administration of endotoxin (bacterial lipopolysaccharides from gram-negative bacteria) to nearly all animal models leads to a rapid and sustained impairment in bile flow. These effects appear to be caused by the release of endotoxin-induced cytokines from resident hepatic macrophages, Kupffer cells, which, in turn, act upon receptors in the sinusoidal membrane of neighboring hepatocytes to induce cell signaling changes that lead to reduced bile formation. The inflammatory milieu causes stimulation of a pro-inflammatory signaling cascade, which leads to reduced expression and activity of a large number of nuclear transcriptional regulators, many of which are essential for maintenance of hepatobiliary transporter gene expression.
It is also likely the endotoxin may act directly upon hepatocytes and cholangiocytes, since these cells have cell surface receptors for endotoxin and other microbial products [71–73]. In addition, the liver is a central player in the hepatic response to infection and injury – the acute phase response – of which one may reasonably include sepsis-associated cholestasis as a component. The hepatic acute phase response is a coordinated transcriptional reprogramming and prioritization of liver function as a means to restore homeostasis and help with injury repair and infection throughout the body. When activated by mediators of inflammation such as endotoxin, the liver changes gene expression to increase secretion of many substances and enzymes to restore homeostasis (e.g., protease inhibitors), fight infection (e.g., complement, C-reactive peptide), and direct amino acids and lipids to the periphery; this is all coordinated via intracellular complex and overlapping cell signaling pathways initiated by endotoxin and cytokines such as tumor necrosis factor-α and interleukins-1β and -6 [74]. These same cytokines that activate the expression of secretory substances from the liver during the acute phase response are also involved in the suppression of function and expression of critical hepatobiliary transporters. Molecular cross-talk between bile acid-activated NRs and pro-inflammatory nuclear mediators provides one means of understanding adaptive processes within the liver.
Drug-Induced Cholestasis
It is well known that many drugs can lead to damage of liver parenchymal cells (e.g., acetaminophen), while some interfere with the basic mechanisms of the formation of bile [75, 76]. Cholestasis as a component of drug-related hepatotoxicity can involve a variety of mechanisms including direct cholangiocyte toxicity and necrosis, impairments in bile acid transport, recruitment of inflammatory cells and thickening of biliary secretions [77].
Infants and young children have reduced detoxification pathways compared with older children and adults, suggesting that there is an enhanced susceptibility to cholestatic effects of certain drugs [78]. This enhanced susceptibility is not fully understood but appears to involve developmentally regulated expression of detoxification and transport genes, immature protective measures against apoptosis/necrosis, and a role for altered inflammatory responses to damaged tissues. With the availability of genome-wide association studies since the mid-1990s, there has been a shift toward their application to personalized components in liver pathobiology. With drug-induced liver injury, the focus is shifting to the interaction between the environment (such as the drug) and genetic correlates and predispositions [79, 80].
Total Parental Nutrition-Associated Cholestasis
Among the more prevalent associations of the rapid progression to end-stage liver disease is the setting of neonates with intestinal failure, who are dependent upon TPN, leading to a condition known as TPN-associated cholestasis. This entity was seen soon after the introduction of TPN in neonates, yet the underlying cause, or more likely causes, are unknown [81, 82]. The typical clinical situation is a premature infant who has damaged or resected small intestine and is unable to advance feeds. Development of TPN-associated cholestasis can occur as soon as two weeks, with hepatomegaly and conjugated hyperbilirubinemia, while cirrhosis has been reported in as little as two months. Moreover, the cholestasis and injury can resolve if patients are weaned off TPN, attesting to the timeliness and confluence of these damaging factors in early infancy. Therefore, there is an inherent susceptibility in this clinical setting that is not readily replicated in older children or adults with TPN dependence.
There appear to be four main contributors to TPN-associated cholestasis: immaturity, infection, inadequate gut function, and a toxic/missing component in the TPN [81]. Despite TPN being administered for nearly 40 years, there is little evidence as to which one of these four contributors are most relevant. Support for all four components has been provided, yet the molecular, or cellular, etiologies remain elusive. The inherent immaturity of bile formation and flow – the “physiologic cholestasis of the newborn” – and drug metabolism pathways support the concept that premature infant livers may be more susceptible to any cholestatic insult, although neither function has been adequately quantified in these babies. The scenario of infection and inflammation contributing to cholestasis in these infants is seen quite often, whereby bouts of infection are often heralded by elevations in serum levels of conjugated bilirubin. Inadequate oral intake reduces the nutritional and hormonal input from the gut to liver function and bile flow, with evidence of an immaturity and impairment of certain gut hormones in intestinal failure and TPN dependence. Finally, many components of the TPN solution have been implicated as cholestatic (minerals, amino acids, sterols, fatty acids to name a few) or absent but, to date, none has been definitively associated with causing cholestasis [83–85]. Recent data cholestatic has identified specific cholestatic components of soy lipids, highlighting the roles of certain phytosterols as pro-inflammatory and FXR antagonists [83, 86–90]. These studies have served as the basis for reducing soy lipid infusions and incorporation of low soy content lipids in the current management of cholestatic neonates on TPN [91, 92].
Adaptation to Cholestasis
In general, the primary location of effecting a response to cholestasis resides within hepatocytes, likely because of this cell’s role in handling bile acids, which can abruptly and profoundly rise in intracellular concentrations with all forms of cholestasis [9]. Reducing the inherent toxicity of retained bile acids within hepatocytes is a major goal of the hepatocyte’s response to cholestasis [50]. When bile acid concentrations rise within cells, there are profound effects on cell signaling and integrity of membranes and subcellular structures. As detergents, bile acids affect membrane fluidity and protein structure, while as cell signaling molecules, bile acids affect kinase pathways, initiate apoptosis, and alter gene expression, among many other critical cellular functions. Over the past few years, the essential components of the hepatocyte’s response to retained bile acids is coordinated to reduce sinusoidal import and synthesis, increase canalicular export, and engage P450-based xenobiotic metabolism pathways (hydroxylation and conjugation) as a means to reduce intracellular concentrations and toxicity. In addition, recent evidence suggests that at least two sinusoidal transporters are activated to export retained bile acids across the sinusoidal membrane (see Figure 3.1). These responses to bile acid overload are, in general, related to bile acids acting as gene regulators – as ligands for several NR family members (mainly CAR, FXR, PXR), which then act as transcriptional activators for target genes whose proteins function to effect the changes noted above (Figure 3.2). This is an evolving avenue of research that overall indicates that the hepatocyte has adaptive responses in place to handle cholestasis, and that these pathways may be amenable to pharmacological therapies [10, 50, 52, 93].
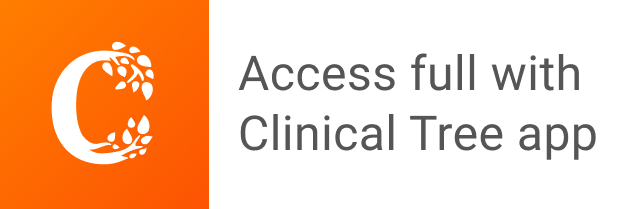