Abstract
Inborn errors of carbohydrate metabolism that lead to hepatic dysfunction are represented mainly by galactosemia, hereditary fructose intolerance (HFI), and glycogen storage disease (GSD) types I, III, IV, VI and IX. Although not related to energy disorders, congenital disorders of glycosylation (CDG) can likewise be placed in this group. The clinical presentation of such patients includes varying degree of hypoglycemia, acidosis, growth failure, and hepatic dysfunction. Appropriate steps in obtaining clinical history, physical examination, and laboratory evaluation support the definitive diagnosis. Advances in biochemistry, molecular biology and genetics continuously increase our treatment options and the development of newer treatment strategies. This chapter highlights our current knowledge.
Introduction
Inborn errors of carbohydrate metabolism that lead to hepatic dysfunction are represented mainly by galactosemia, hereditary fructose intolerance (HFI), and glycogen storage disease (GSD) types I, III, IV, VI and IX. Although not related to energy disorders, congenital disorders of glycosylation (CDG) can likewise be placed in this group. The clinical presentation of such patients includes varying degree of hypoglycemia, acidosis, growth failure , and hepatic dysfunction. Appropriate steps in obtaining clinical history, physical examination, and laboratory evaluation support the definitive diagnosis. Advances in biochemistry, molecular biology and genetics continuously increase our treatment options and the development of newer treatment strategies. This chapter highlights our current knowledge.
Disorders of Galactose Metabolism
In 1935, Mason and Turner provided the first detailed characterization of a galactose-intolerant individual [1]. Since then, classically, three distinct disorders of galactose metabolism and several variant forms of the disease have been described. More recently a fourth subtype has been discovered in Asia [2]. These disorders are transmitted by autosomal recessive inheritance and are expressed as a cellular deficiency of one of the enzymes in the metabolic pathway through which galactose is converted to glucose: galactose-1-phosphate uridyl transferase, galactokinase, uridine diphosphate (UDP) galactose-4-epimerase and the newly discovered galactose mutarotase. Since each of these conditions results in milk-induced galactosemia but represents distinct biochemical entities, the terms transferase-deficiency galactosemia, galactokinase-deficiency galactosemia, and epimerase-deficiency galactosemia have traditionally been used to distinguish between the three main forms of the disease (galactosemia types I–III respectively). Each of these enzymatic defects associated with galactosemia results in a distinctive clinical presentation. Clinical manifestations of toxicity in transferase-deficiency galactosemia, the classic form of the disease (type I), include progressive lethal neonatal liver disease, malnutrition, growth failure, cataract formation, mental retardation, and ovarian failure [3]. Galactokinase deficiency (type II), originally described by Gitzelmann in 1967, results primarily in cataract formation and galactosuria [4]. In most cases of UDP-galactose-4-epimerase deficiency (type III), the defect is limited to erythrocytes and leukocytes; therefore, affected individuals display no clinical or laboratory manifestations of galactosemia [5]. In a variant form of epimerase deficiency galactosemia identified by Holton and colleagues in 1981, however, the defect is more generalized and results in a severe clinical presentation resembling the classic form of the disease [6]. Galactose mutarotase deficiency (type IV) presents similarly to galactokinase deficiency. It was only recently described in eight Japanese patients [2]. Finally a few other disorders can also lead to elevated blood galactose levels such as porto-systemic shunts, citrin deficiency and Fanconi-Bickel syndrome.
Treatment of classic galactosemia has remained essentially unchanged since the disorder first was described more than 60 years ago. Confidence in dietary strategies that effectively minimize galactose intake in affected individuals, however, has declined significantly since the late 1990s. This coincides with recognition that long-term complications such as learning difficulties, speech disorders, ovarian failure, and ataxia syndromes commonly occur in well-treated patients. It is now clear that development of new treatment strategies is necessary to positively impact the ultimate outcome of this disorder. To this end, future research efforts should be focused on developing a complete understanding of the molecular and biochemical basis of galactosemia, particularly as they relate to the pathogenesis of these long-term complications [7].
Biochemistry of Galactose Metabolism
Galactose is a monosaccharide that is derived from the hydrolysis of lactose, the sugar in dairy products. Lactose is hydrolyzed into glucose and galactose by the disaccharidase lactase in the brush border membranes of the enterocytes. Galactose is transported across the brush border membrane of the enterocyte through the sodium-dependent glucose–galactose transporter [8]. Galactose is metabolized to glucose in a series of reactions as depicted in Figure 27.1. In solution, galactose exists in equilibrium between the α- and β-anomers. Although the two anomers do spontaneously interconvert in aqueous solution, the rate of utilization of α-D-galactose can exceed its rate of generation. Therefore, galactose mutarotase (aldose 1-epimerase, GALM) catalyzes this reaction [9].
Figure 27.1 Galactose metabolism.
The first real step in galactose metabolism involves phosphorylation of the α-anomer D-galactose by ATP utilizing the enzyme galactokinase. Galactokinase in the human liver shows developmental changes, with progressive increase from the seventh week of gestation until term [10]. The level of activity in the red blood cells is higher in the newborn than in the adult. The enzyme, however, is not regulated by galactose.
The second step in galactose metabolism involves the reaction of galactose 1-phosphate with UDP-glucose, which is catalyzed by the enzyme galactose-1-phosphate uridyltransferase. The enzyme is present in most mammalian tissues including the liver. This reaction results in the formation of UDP-galactose and glucose 1-phosphate.
The third step in galactose metabolism involves the interconversion of UDP-galactose to UDP-glucose catalyzed by UDP-galactose-4-epimerase.
Alternative pathways of galactose metabolism (Figure 27.2) are used in case of metabolic blocks in the pathway. These involve reduction to galactitol through two enzymes: aldose reductase and L-hexonate dehydrogenase. The existence of this alternative pathway explains the presence of galactitol in the urine of patients with both transferase and galactokinase deficiencies. The other pathway involves oxidation of galactose to galactonate. Patients with kinase or transferase deficiency excrete galactonate in their urine. A final pathway (pyrophosphorylase) uses the UTP-dependent glucose/galactose pyrophosphorylase to produce glucose-1-P. This pathway is believed to be responsible for endogenous galactose production [11].
Figure 27.2 Alternative pathways of galactose metabolism.
Humans are capable of metabolizing large quantities of galactose, as evidenced by the rapid clearance of galactose from blood [12]. Elevation of plasma glucose occurs shortly after galactose infusion as a result of conversion of galactose to glucose. Tracer studies indicate that as much as 50% of galactose may be found in glucose pools within 30 minutes of injection [12]. The removal mechanism of galactose from the blood is saturated at plasma levels of about 50 mg/dL secondary to the limited ability of galactokinase to phosphorylate the sugar [13]. When blood levels increase by 30–40 mg/dL, urinary losses become substantial [13]. During infancy, 40% of calories are derived from the hydrolysis of lactose to galactose and glucose. Therefore, the conversion of galactose to glucose is of importance to maintain normoglycemia, and enzymatic defects of this pathway are most likely to produce clinical signs and symptoms as well as marked elevations of blood and urine galactose levels during a crucial period of development.
Transferase-Deficiency Galactosemia (Galactosemia Type I)
The first described defect leading to galactosemia results from deficient activity of the enzyme required for the second main step in galactose metabolism (Figure 27.1). The consequences of this defect are much more severe than those of the other three defects, galactokinase deficiency, UDP-galactose-4-epimerase deficiency and galactose mutarotase deficiency, with prominent hepatic involvement.
Molecular Basis of Transferase-Deficiency Galactosemia
Transferase deficiency is an autosomal recessive disorder. The sequences of the homologous proteins from Escherichia coli, from Saccharomyces cerevisiae, and from humans have been reported and show overall sequence identity of 35% [14]. The cDNA encoding the human transferase enzyme is 1,295 bases in length and predicts a 43 kDa protein [15]. The gene (GALT) has been mapped to chromosome 9p18, spans 4 kb, and has 11 exons. The amino acids histidine (164)–proline–histidine (166) form an active site sequence that is essential for activity of the enzyme [16]. Southern, northern, and western blot experiments suggested that the majority of the patients with galactosemia have missense mutations that result in low or undetectable enzymatic activity [17]. So far more than 300 mutations have been identified [18] and can be found in an online database available at: www.arup.utah.edu/database/GALT/GALT_welcome.php .
The two most commonly characterized mutations lead to glutamine 188 substitution by arginine (Q188 R) and lysine 285 by asparagine (K285 N), which account for 75% of all mutations in Caucasian and Hispanic populations [19]. Substitution of arginine 333 by tryptophan (R333 W) occurs at a highly conserved domain in the homologous enzymes from E. coli, yeast, and humans. Several other mutations have been described, such as valine 44 to methionine (V44 M) and methionine 142 to lysine (M142 K). Therefore, it appears that transferase-deficiency galactosemia results from missense mutations that tend to occur in regions that are highly conserved throughout evolution while polymorphisms occurring in non-conserved domains result in normal enzymatic function [20].
Leucine substitution by serine (L135S) occurs mostly in African-Americans. Homozygosity for this pathogenic variant causes “clinical variant galactosemia.” These individuals have some aspects of classic galactosemia (such as life-threatening liver disease) but do not have the long-term complications associated with classical galactosemia [21].
Asparagine substituted by aspartic acid (N314D) that occurs in Caucasians, Asians as well as African-Americans and is in combination with the (c.-119_-116del) the basis for the Duarte variant. This variant is benign as the transferase expresses diminished but adequate enzyme activity [22]. No treatment is required for this variant [23].
Clinical Presentation
Since its first description in 1935, numerous patients with transferase-deficiency galactosemia have been followed for years and reports of variable clinical presentations, growth, and developmental patterns, and long-term prognosis have been published. These reports, coupled with case descriptions published since 1935, have established clearly the clinical entity of this disease.
Currently most patients are detected through newborn screening.The paradigmal presentation of this disease in countries where there is no newborn screening starts with vomiting or diarrhea (present in 95% of patients), anorexia, and hypoglycemia in the first few days after the initiation of milk feeding. This will evolve to neonatal cholestasis, but as there can also be hemolysis, apart from the direct bilirubin, a respectable fraction can be unconjugated. When a dietary treatment with exclusion of galactose is not initiated promptly, this will rapidly deteriorate to neonatal acute life-threatening liver failure and/or a sepsis. Typical for the liver failure is that transaminases can be relatively mildly elevated despite the presence of an INR >1.5–2 and hypoglycemia is more prevalent. There can be bruising or hepatomegaly. Ascites may develop within two to five weeks after birth, as a result of continued galactose ingestion and is present in most infants who succumb to the disease.
In 1977, Levy and associates identified a direct correlation between galactosemia and neonatal E. coli sepsis. In their review of over 700,000 infants screened during a 12-year period, four of eight infants were diagnosed with septicemia and transferase-deficiency galactosemia during the second week of life; three of the four died [24]. Thirty-five more patients with classic galactosemia were identified through further review of data from routine screening of over 2.5 million infants from eight other US states. E. coli sepsis was documented in ten of the 35 patients, and nine of these died despite antibiotic therapy. Systemic infection seems to develop at approximately 7 to 14 days of age and appears to be directly associated with continued galactose ingestion secondary to inhibition of leukocyte bactericidal activity by the sugar [25]. As a result of these important clinical observations, neonates diagnosed with galactosemia or E. coli sepsis should undergo further evaluation to rule out the alternative condition.
In milder cases, moderate intestinal upset after galactose ingestion may be the only manifestation. Failure to thrive is nevertheless the most common presenting symptom and occurs in almost all patients. Additionally, a few individuals have been found to be entirely asymptomatic on milk feedings. These patients have “clinical variant galactosemia” [26]. As lactose-free formulae have become increasingly accessible (e.g., soy/rice-based), and feeding trials with these products often are employed in infants who experience recurrent vomiting and growth failure early in life, a child with galactosemia may display improvement in symptoms without recognition of the underlying defect. In such patients, galactosemia may remain undetected through the first several months of life until motor retardation, hepatomegaly, or cataracts develop [27]. Still others may be diagnosed after several years of life. These individuals usually suffer from mental retardation and visual disturbances caused by cataracts and frequently have a history of vomiting after milk intake managed by reduced intake or use of milk substitutes [27].
Cataracts can develop early within the postnatal period, or they may be present at birth if the mother ingested generous amounts of dairy products late in pregnancy. These punctate lesions in the nucleus of the lens may be so small that slit-lamp examination is required for visualization. Signs of increased intracranial pressure and cerebral edema also have been observed as a presenting feature [28]. Mental retardation may become apparent after several months.
Laboratory Findings
Aberrant laboratory findings may be varied but include elevated blood and urinary levels of galactose (cross-reacting with glucose on urinary strip testing), hyperchloremic acidosis, albuminuria, aminoaciduria, hypoglycemia (or hyperglycemia if galactose cross reacts on point-of-care testing devices with glucose dehydrogenase-based systems using pyrroloquinoline quinone), and blood changes reflecting deranged liver function (with deranged INR, relatively mild elevation of transaminases and often a mixed hyperbilirubinemia) . Occasionally, infants may have severe and prolonged hypoglycemia. It apparently is caused by the inhibition of glucose release from glycogen [29]. In addition there is an inhibition of glucose formation through gluconeogenesis [30], likewise galactose is an important source of energy for the neonate. The galactosuria may be intermittent because of poor food intake or may disappear within three or four days of intravenous feeding. Therefore, if the urine is not tested for reducing sugars during a period of galactosuria, (such as a few hours after a meal), the diagnosis may not be suspected. The finding of a urinary reducing substance that does not react with the glucose oxidase test should be suspicious for galactosemia. This finding does not establish the diagnosis, because several other conditions such as fructosuria, lactosuria (from deficient intestinal lactase), and severe liver disease of any origin may impair the clearance of blood galactose and result in the presence of urinary reducing sugar that is not glucose [27].
Elevation of plasma amino acids such as phenylalanine, tyrosine and methionine as markers of liver dysfunction can be present and erroneously lead to a diagnosis of tyrosinemia.
Further tests that are indicative for galactosemia are discussed below, see “Diagnosis.”
Biochemical Features and Pathogenesis of Toxicity
Pathologic changes that accompany galactosemia affect the liver, lens of the eye, brain, and kidney. Toxicity seems to result primarily from accumulation of two by-products of galactose metabolism, galactose 1-phosphate and galactitol (Figure 27.2). The biochemical causes of toxicity in individual organs may differ, depending on the metabolic patterns and functions of the involved organs.
Liver
Hepatic changes associated with transferase-deficiency galactosemia result entirely from abnormal galactose metabolism. In affected individuals, galactose ingestion results in elevated levels of both galactose 1-phosphate and galactitol in the liver. Other findings, however, suggest that one or more additional metabolites act alone or together to produce the liver damage seen in this form of the disease. For example, liver damage does not occur in normal laboratory animals fed diets rich in galactose, despite hepatic galactitol accumulation in chicks [31] and hepatic accumulation of galactose 1-phosphate in rats [32]. Furthermore, humans with galactokinase deficiency accumulate large amounts of galactitol but develop no liver damage. The amount of galactosamine, which is known to stimulate hepatocellular changes in animals, was found to be increased in one patient with galactosemia [33]. Other described mechanisms are NADPH depletion through galactitol formation, phosphate depletion and the formation of galactonate [11]. Nevertheless, the cause of the entire toxicity syndrome in transferase deficiency remains uncertain.
Kidney
Individuals with transferase-deficiency galactosemia develop renal tubular dysfunction following galactose ingestion; over time, levels of galactose 1-phosphate and galactitol accumulate in the kidneys [33]. Alteration in kidney function appears to result primarily from increased galactose 1-phosphate levels, because patients with galactokinase deficiency who characteristically excrete large amounts of galactitol do not develop renal impairment. Galactose 1-phosphate accumulation also may produce the aminoaciduria seen in this disorder through secondary inhibition of amino acid absorption by the tubules [34]. The inhibition is non-competitive and similar to that seen in human intestine [35].
Lenticular Changes
The specific mechanisms for cellular changes in the eye are more clearly understood than in other organs. Changes in the lens seem to result primarily from galactitol accumulation, which initially was reported by van Heyningen in 1959 [36]. Later, Kinoshita and colleagues demonstrated that a concomitant increase in water content occurred with galactitol build-up caused by oncotic pressure exerted by the alcohol [37]. Poor diffusion of galactitol from these tissues leads to further damage and cataract formation. Application of an osmotically balanced incubation medium prevents opacification [37].
Nutrient supplementation has been shown to alter the rate of cataract formation in animals. Biochemical changes induced by galactose feeding include decreases in several enzymatic reactions, amino acid transport, protein synthesis, and alterations in ion fluxes. These occur simultaneously as cataract formation takes place [37–39]. As little as two days of galactose ingestion can reduce glycolysis and lenticular respiration by approximately 30%, and this reduction is sustained until cataracts are formed. Nutrient imbalances and changes in lenticular water content resulting from galactitol accumulation are principal initiators of lenticular opacification.
Brain
Galactitol accumulates in higher concentrations in brain tissue of humans and rats fed galactose than in any other tissue except the lens [32]. Therefore, galactitol appears to be a factor in the development of brain function abnormalities seen in transferase-deficiency galactosemia. On the other hand, damage from galactitol accumulation in patients with galacto-kinase deficiency seems to be limited to the lens.
Pathologic alterations in brain tissue of individuals with transferase-deficiency galactosemia may not be completely reversible through dietary galactose restriction. Considerable attention has been paid to defining the specific mechanism for galactose-induced brain damage. Studies in chick brain showed that galactose administration diminished ATP, reduced brain glucose and glycolytic intermediates, redistributed hexokinase, enhanced fragility of neural lysosomes, and decreased fast axoplasmic transport [40, 41]. The effects could be temporarily reversed by glucose [42]. Changes in the chick brain appear to be related to several factors such as hyperosmolality, alterations in energy metabolism, abnormal serotonin levels, and interference with active uptake of glucose into the neurons [40, 41, 43]. It remains to be determined whether these changes in chicks are similar to galactose-induced abnormalities in patients with transferase deficiencies.
Gonads
Among classic galactosemic females, 90% have ovarian failure, which is present shortly after birth. Biochemically, follicular-stimulating hormone is high whereas antimullerian hormone is low compared with controls (hypergonadotropic hypogonadism) [44]. Males with galactosemia have normal testicular function. The mechanism underlying ovarian failure in galactosemic women is not known, although galactose toxicity has been implicated. Despite the documented ovarian failure in most of the affected patients, successful pregnancies have been reported [45].
Pathology
Early hepatic lesions, present in the first weeks of life, consist of cholestasis and diffuse fatty vacuolation with little to no inflammatory reaction. The fatty changes are extensive and generalized throughout the lobule. Later, disorganization of the liver cells with pseudoductular and pseudoglandular formation occurs. This tendency toward pseudoglandular orientation of cells has been described as characteristic of galactosemia but is relatively non-specific. As the disease progresses, delicate fibrosis appears, first in the periportal regions and eventually extending to bridge adjacent portal tracts. Regenerating nodules and hepatic fibrosis are late features that, with continued galactose ingestion, progress to cirrhosis similar in many respects to the cirrhosis of ethanol abuse. Death usually occurs in the first year of life unless galactose intake is decreased or curtailed. Frank cellular necrosis is unusual but may occur with large amounts of dietary galactose. Despite the severity of the hepatic lesion, there is a remarkable lack of infiltration of inflammatory cells [46].
Except for cataract formation in the lens, other tissues show only minor changes. Kidneys show dilation of tubules at the corticomedullary junction. The spleen enlarges as a result of portal hypertension. Lesions in the brain are subtle, with minor loss of nerve cells and gliosis in the dentate nucleus and gliosis in the cerebral cortex and gray matter [47].
Diagnosis
The presence of urinary reducing sugar that does not react with glucose oxidase reagents in an infant with vomiting and growth failure on milk feedings supports a presumptive diagnosis of galactosemia. During the first two weeks of life, some normal premature and term infants excrete up to 60 mg/dL of galactose in urine. Furthermore, it should be remembered that lactose, fructose, and pentose can produce the same urine test result, and that the specific sugar may be identified only by paper or gas–liquid chromatography. Paper impregnated with galactose oxidase makes screening for galactosuria easier. Regardless, galactose restriction should be instituted promptly awaiting further results in patients with cholestasis or liver failure – even if this means that breastfeeding is temporarily halted. Galactitol, formed through the alternative pathways, can also be measured in urine.
Confirmation of the diagnosis should be made through direct measurement of transferase activity. Xu and colleagues developed a highly sensitive radiochemical assay that can detect galactose 1-phosphate uridyl transferase activity as low as 0.1% of normal in erythrocytes and leukocytes [48]. Measurement of red cell UDP-glucose consumption has been employed extensively as a diagnostic test for galactosemia since the late 1980s [27]. It is based on quantification of UDP-glucose before and after incubation of galactose 1-phosphate using added red cell hemolysate as the enzyme source. Results are obtained through spectrophotometric measurement of nicotinamide adenine dinucleotide (NAD), which is formed from hepatic NAD through the conversion of UDP-glucose to UDP-glucuronic acid by UDP-glucose dehydrogenase. Homozygous patients exhibit complete absence of red cell transferase activity. Heterozygous carriers typically display intermediate levels of enzyme activity. Infants with 50% of normal enzyme activity should undergo further tests to identify the presence of a specific variant of the disease [49]. There is a clear link between the genetic pathogenic variants, global residual enzyme activity and clinical phenotype.
Care should be taken that enzymatic testing on RBCs can only be performed in the absence of blood transfusion, as false-negative results are possible up until four months post-transfusion. Galactose tolerance tests should never be employed for this purpose, because it has been suggested that a single exposure to a large quantity of galactose may produce brain injury resulting from prolonged severe hypoglycemia.
Genetics
Population studies indicate that the incidence of heterozygosity for galactosemia is between 0.9–1.25% and that between 8–13% carry the Duarte gene [50]. Incidences of transferase-deficiency galactosemia derived from large-scale screening in neonatal nurseries have been between 1:10,000 and 1:70,000 live births [51].
With the advent of screening for galactosemia, multiple variants of this disease have become apparent, the variants being more prevalent than classic transferase-deficiency galactosemia [49]. There are three homozygotic types.
1. “Classic” galactosemia is autosomal recessive (e.g., Q188 R/Q188 R, K285 N/K285 N, L195P/L195P, etc. [21]), and there is no transferase activity in erythrocytes, fibroblasts, liver, and presumably in any other tissue. In heterozygotic, unaffected carriers, activity is 50% of normal. The Q188 R/Q188 R is the most common genotype in Caucasians and is associated with a less favorable long-term outcome.
2. The Duarte variant (4bp 5′ del + N314D/Q188 R) is the most common form of galactosemia and is only detected by enzymatic screening, as these infants are asymptomatic. Red cell transferase activity is 50% of normal, and on starch gel electrophoresis the enzyme migrates faster than normal. This does not require treatment [23].
3. In the clinical variant galactosemia (S135L/S135L), erythrocytic transferase activity is absent, but 10% of normal activity is present in liver and intestine. Patients with this variant may also develop a galactose toxicity syndrome in the neonatal period.
All pathogenic variants are collected in an online database which can be found at: www.arup.utah.edu/database/GALT/GALT_welcome.php .
Screening for Galactosemia
The rationale for genetic screening is three-fold: (1) to detect disease at its incipient stage and thereby offset harmful expression of the mutant genotype through appropriate medical treatment; (2) to identify a variant genotype for which reproductive options (family planning) may be provided; and (3) to identify gene frequency or biologic significance and natural history of variant phenotypes.
Various screening methods for galactosemia have been used [52]. The original Guthrie test used filter-paper blood samples from which a microbiologic assay detected elevated galactose levels. The newer Beutler test assays the erythrocyte transferase activity directly from dried filter paper, and the Paigen assay is an improved bacteriologic method that includes detection of elevated levels of galactose and galactose 1-phosphate. Measurements of elevated galactose require that the infant receive sufficient dietary galactose or a false negative test will result. Conversely, the normal enzyme may become inactive in a hot or humid climate, and a false positive (negative enzyme activity) may be reported [53].
In all of the states of the USA newborn screening for classical galactosemia is performed [53]. In Europe, there is a more diverse picture (screening in Austria, Germany, Hungary, Ireland, Sweden, Switzerland, The Netherlands and as a pilot program in Turkey, Italy and Belgium) [11].
In utero assay for galactosemia is indicated in pregnant women with a family history of galactosemia. Cultured fibroblasts from amniotic fluid can be assayed for transferase activity. Additionally, the technique of chorionic villus sampling has been used to detect galactosemia during the tenth week of gestation [54]. Cloning of the cDNA encoding for the transferase enzyme and the finding that the majority of galactosemic patients have missense mutations have allowed for rapid molecular approaches using the polymerase chain reaction to detect common mutations.
Treatment
Although the cause of the entire toxicity syndrome in transferase deficiency is uncertain, there is no disagreement that elimination of galactose intake reverses the biochemical manifestations of transferase-deficiency galactosemia. Some patients seem to have increasing tolerance to galactose with advancing age; however, studies using [14C]-galactose do not support the clinical impression that alternative pathways of galactose metabolism develop at puberty, nor is there any indication that any drug will increase galactose oxidation, although some patients with variant forms of transferase deficiencies can oxidize limited amounts of galactose [26, 27].
The only acceptable treatment at present is elimination of dietary galactose [12, 23, 55]. Preparations used in treating infants are Pregestimil, Nutramigen, and the soybean milk preparations. Both Pregestimil and Nutramigen are prepared from casein and may contain small amounts of lactose, but this amount of lactose does not appear to be sufficient to impair therapeutic efficacy. The soybean formulae contain small amounts of galactose in raffinose and stachyose, and other dietary constituents contain small amounts of galactosides, but these carbohydrates are not digested by human intestinal enzymes and should not affect the efficacy of treatment [55]. Another possible alternative is a rice-based infant formula.
Despite initial optimism in treatment success with full recovery from the typical neonatal disease (and acute liver failure) it became evident that long-term complications still occur despite lifelong “optimal” dietary regimen. In fact, many of the long-term complications correlate inversely to the strictness of the diet [56]. Currently, after an initial period of complete restriction and a lifelong diet that eliminate the sources of lactose and galactose from dairy products, small amounts of galactose from non-dairy products (for instance tomatoes, legumes) and some specific mature cheeses (with galactose content <25 mg/100g) are allowed [23]. It is possible that some input of galactose is required to have normal protein glycosylation and there will always be endogeneous production of galactose [11].
It is important to be aware that asymptomatic heterozygotic mothers may have elevated serum galactose levels after ingestion of diets high in milk. Infants delivered of such mothers may have the galactosemic syndrome at birth. For this reason, restriction of galactose during the pregnancies of women who have previously borne children with galactosemia is recommended [26, 27, 55]. The use of uridine and aldose reductase inhibitors in galactosemic patients has not been shown to be effective despite their theoretical advantage [57, 58]. Boxer et al. [59] have been able to generate a small molecule capable of selectively inhibiting galactokinase thus preventing conversion of galactose to galactose 1-phosphate. This has not been tested in vitro or vivo yet.
Given the dietary restrictions, care should be taken to maintain normal calcium intake and adequate 25-OH-vitamin D levels.
Prognosis
When untreated, galactosemia results in early deaths of many affected children and is attended by the prospect of mental retardation of those who survive. In a series of 43 galactosemic patients, there were 13 neonatal deaths, which occurred at an average age of six weeks and were usually attributed to infection [55]. Levy and co-workers noted that nine of 35 patients died of E. coli infections and strongly recommended early cultures and institution of antibiotics effective against E. coli in any infant with galactosemia who appears ill [24].
Treatment of galactosemic patients with a galactose-free diet results in survival with reversal of the acute symptoms, normal growth, and complete recovery of liver function; however, the long-term outcome is not entirely normal. Experience gained in the long-term observation data of 509 patients in the GalNet registry [11] show that most affected patients experienced neonatal manifestation (79%) and despite following a diet developed brain impairments (85%), primary ovarian insufficiency (79%) and diminished bone mineral density (26%).
Brain impairments consisted of global developmental delay (52%). The great majority of this group had likewise language delay (78%). Moreover, isolated language delay was present in 28%. Neurological complications were present in 52% with tremor being the most common in 31% followed by motor abnormalities (clumsiness, coordination difficulties), ataxia, seizures and dystonia. Mental and behavioral problems occurred in 44% (anxiety disorders, depression, ADHD). Diagnosis through newborn screening and the initiation of dietary therapy within the first week of life were positive modifiers. Evidence indeed supports the previous impression that a more favorable outcome can be expected when a patient is treated at an early age. For example, the mean IQ of 16 patients treated before seven days of age was 99.5, whereas that of patients treated between four and six months of age was 62. It is generally desirable to institute treatment at the earliest possible age, and neonatal screening is an important step in this direction. On the contrary too strict diet (restriction in fruit and vegetables as opposed to only restriction of dairy) and <1% enzyme activity predicted neurological complications and higher occurrence of mental and behavioral problems respectively [11].
While in boys there were no endocrine disturbances, in female patients spontaneous puberty was only reported in 51%. Premature ovarian insufficiency was present in 79%. Nevertheless, of these women with premature ovarian insufficiency who attempted to get pregnant, 25% were successful.
Osteoporosis is a frequent complication, certainly among females with galactosemia. The mechanisms underlying this complication may relate to low calcium intake, lack of sex hormones associated with ovarian failure, and an independent defect in collagen synthesis resulting in disturbances in bone mineralization [60].
Galactokinase-Deficiency Galactosemia (Galactosemia Type II)
Galactokinase deficiency is less common than classic transferase deficiency, with an incidence of about 1 in 10,000 [27]. It does not result in progressive liver disease and mental retardation, but galactose exposure may result in cataract formation [4]. It is appropriate to compare this entity with transferase deficiency because it affects the first reaction (kinase) and the transferase is the second reaction of the galactose pathway (Figure 27.1). Comparison of patients with these defects and those with a defect involving the third reaction (epimerase) has helped to define some of the mechanisms of toxicity in several organs, including the development of cataracts. With galactokinase deficiency, there is no accumulation of galactose-1-phosphate, and usually no systemic manifestations. Cataract formation is related to synthesis of galactitol in the lens and osmotic disruption of lens fiber architecture, as discussed above (Figure 27.2). An early start of a galactose-restricted diet resulted in regression or prevention of cataracts [61], but slight cataracts without visual impairment occurred in 50% of the patients, 56% of whom were non-compliant. Clinical symptoms of hypoglycemia, mental retardation, microcephaly, and failure to thrive, were associated with non-compliance [61]. Maternal galactokinase deficiency may result in fetal cataract formation [27]. Because of the potential for cataract formation, lifelong elimination of galactose is suggested. The gene (GALK1) has been mapped to chromosome 17q24, and 20 mutations have been described in the gene for galactokinase, resulting in loss of the activity of the enzyme [62]. There is no liver disease in galactokinase deficiency.
Galactose Epimerase-Deficiency Galactosemia (Galactosemia Type III)
Galactose epimerase catalyzes the third reaction of galactose metabolism (Figure 27.1). Epimerase deficiency was discovered incidentally while screening for galactosemia and has an incidence of about 1 in 46,000 in Switzerland. Patients have normal erythrocyte transferase activity but elevated levels of galactose 1-phosphate [5]. One form of this condition is apparently caused by a decreased stability of the epimerase and leads to enzyme deficiency in those cells in which its turnover is slow or absent, such as erythrocytes [62]. It is, therefore, considered to be a benign illness in as much as the enzyme deficiency is limited to leukocytes and red blood cells. Affected people with the form limited to leukocytes and red blood cells have no symptoms. However, patients with generalized epimerase deficiency have been described and these patients have signs and symptoms identical to transferase-deficiency galactosemia, including the neonatal life-threatening liver failure [6].
In contrast to transferase deficiency, in which UDP-galactose can be formed from UDP-glucose, one patient with generalized epimerase deficiency was unable to synthesize the galactose precursor necessary for synthesis of glycoproteins and glycolipids. These glycosylated compounds are necessary for cell membrane integrity, particularly in the central nervous system. Therefore, in contrast to patients with transferase deficiency, the rare patient with systemic epimerase deficiency may require small quantities of galactose for normal growth and development. One patient with epimerase deficiency continued to show slightly elevated levels of galactose 1-phosphate in red cells even with dietary restriction of galactose. Appropriate treatment of this disorder, therefore, requires frequent monitoring of erythrocyte galactose 1-phosphate levels in order to best determine the optimal dietary level of galactose.
Galactose Mutarotase-Deficiency Galactosemia (Galactosemia Type IV)
Galactose mutarotase deficiency has only been described in one recent article [2]. In eight patients from Asian descent, trio-based exome sequencing could demonstrate this enzyme to be responsible for the unexplained type of galactosemia. While most patients were asymptomatic, two patients had cataracts. Elevated galactose levels were documented and the three other types of galactosemia as well as porto-systemic shunts, other cholestatic diseases (e.g., citrin deficiency) or Fanconi-Bickel syndrome were excluded. Genetic analysis revealed biallelic pathogenic variants in galactose mutarotase suggesting the presence of a novel type of galactosemia, namely, type IV galactosemia. While non-enzymatic conversion of β-D-galactose to α-D-galactose occurs spontaneously in water, galactose mutarotase is necessary for normal cell growth at least in some organisms. Particularly during the lactation period when infants receive large amounts of galactose it is possible that this enzymatic action is needed. Moreover, after the start of a galactose-restricted diet, the congenital cataracts disappeared. As most patients were asymptomatic, the diagnosis will most often be made in countries where there is newborn screening for galactosemia. Overall the phenotype is similar to galactokinase deficiency [2].
Disorders of Fructose Metabolism
There are three recognized disorders of fructose metabolism. Until the mid-1950s, the only identified defect was the benign disorder essential fructosuria [63]. This results from fructokinase deficiency, which converts fructose to fructose 1-phosphate. It was first revealed in a patient checked for glycosuria while being investigated for possible diabetes [64]. Fructose, although containing no aldehyde group, becomes a reducing sugar in basic solution and will give a positive urine test for reducing substances but a negative reaction on glucose oxidase testing.
In 1956, it was noted in some patients that ingestion of fructose was followed by vomiting, severe hypoglycemia, and liver disease [65]. A year later, this illness was characterized and named hereditary fructose intolerance and was found to be due to a deficiency in fructose-1-phosphate aldolase (adolase B) [66]. A third disorder of fructose metabolism, caused by fructose-1,6-diphosphatase (FDPase) deficiency was identified in 1970. It was associated with fasting-induced as well as diet-induced hypoglycemia, but more strikingly, both fasting and dietary fructose caused lactic acidosis [67]. These three disorders are distinct both clinically and biochemically. Essential fructosuria does not cause liver injury. Patients with FDPase deficiency may show transient fatty infiltration of the liver. In contrast, liver injury may be a significant feature of HFI.
Biochemistry of Fructose Metabolism
Fructose is a monosaccharide that belongs to the ketose group and is a widely distributed compound in nature. Free fructose is found in fruits and in honey. A major source of fructose is the disaccharide sucrose, which is hydrolyzed into fructose and glucose by the disaccharidase sucrase at the brush border membrane of enterocytes. Fructose is transported across the intestinal and liver plasma membranes via a carrier protein called GLUT5, a sodium-independent transporter [68]. Another hidden source of fructose is sorbitol which is dehydrogenated to fructose. Once absorbed, fructose is utilized mainly by the liver, kidney, and small intestine. Approximately 75% of fructose is taken up by the liver; the kidney and small intestine take up the remaining 25%. These tissues possess specialized enzymes involved in fructose metabolism. These enzymes are fructokinase, aldolase B and triokinase (Figure 27.3).
The first step in fructose metabolism involves fructokinase, which catalyzes the phosphorylation of fructose to fructose 1-phosphate. The next step involves fructoaldolase. Three aldolase isozymes have been identified. Aldolase B is present in the liver, kidney, and small intestine and acts on fructose 1-phosphate to produce D-glyceraldehyde and fructose-1,6-diphosphate to produce dihydroxyacetone phosphate. The two other aldolases are aldolase A, found in the muscle, and aldolase C, found in the brain. Both aldolase A and C have much greater activity against fructose-1,6-diphosphate than aldolase B. The presence of these two enzymes allows gluconeogenesis and glycolysis to continue even in the absence of aldolase B. The cDNA encoding for human and rat aldolase B has been cloned [69] and the gene is localized to chromosome 9q22.3 [70]. The resulting D-glyceraldehyde is converted into D-glyceraldehyde 3-phosphate by triokinase. Glyceraldehyde-3-P is part of the glycolysis and gluconeogenesis pathway.
Other enzymes involved in fructose metabolism include fructose-1,6-biophosphatase, which catalyzes the splitting of fructose-1,6-diphosphate to fructose 6-phosphate and phosphate. This process is irreversible but the reverse reaction is catalyzed by phosphofructokinase. Alternative pathways of fructose metabolism involve the conversion of fructose directly to fructose 6-phosphate by hexokinase and glucokinase. The affinity of these two enzymes for fructose, however, is several-fold lower than that for glucose, hence the origin of the fructosuria in fructokinase deficiency.
Hereditary Fructose Intolerance (Aldolase B Deficiency)
In 1956, Chambers and Pratt described HFI in a young woman who complained of vomiting after ingestion of fruit or sugar [71]. The authors recognized the variation in symptoms from those of essential fructose-uria and speculated that the illness resulted from accumulation of a toxic intermediate. In 1957, Froesch and associates [72] reported the syndrome in two siblings and two relatives and proposed that aldolase deficiency was the causative factor, based on the results of two liver biopsies. The defect was later characterized as an inability of aldolase to split fructose 1-phosphate [73].
Molecular Basis
The frequency of HFI is 1 in 20,000 individuals. It has a recessive mode of inheritance and is caused by a deficiency of aldolase B, which is normally present in the liver, kidneys, and small intestine. The enzymatic activities of aldolase A in muscle and aldolase C in brain are normal [74]. The activity of tissue aldolase B is reduced to less than 15% of normal values. The three isoenzymes are related and are derived from a single ancestral gene.
The aldolase B gene (ALDOB) has been sequenced and is mapped to human chromosome 9q13-q32 [75]. The gene has 14,500 bp, nine exons, and encodes 364 amino acid residues [75]. The first mutation described was a G to C change in exon 5, which resulted in alanine substitution by proline at position 149 of the protein (A149P) within a region critical for substrate binding. The G to C transversion created a new recognition site for the restriction enzyme Aha II [76]. The alanine at position 149 is a conserved amino acid because it is present in aldolase B of humans, rats, and chickens [77]. The substitution of proline is likely to disrupt the spatial configuration of juxtaposed residues in aldolase B and adversely affect its catalytic activity. The mutation resulting in A149P was found in 33 of 50 patients (67%) with HFI [78]. The mutation is encountered more frequently in patients from northern than from southern Europe. Several other mutations have been described, such as that leading to alanine substitution by aspartic acid at position 174 (A174D) and asparagine substitution by lysine at position 334 (N334 K) [79]. The molecular defects in aldolase B alleles were characterized in 31 North Americans with HFI [80]: 59% had A149P, 11% had A174D, and 2% had N334 K. So far, more than 45 disease-causing mutations have been reported. The missense mutations could be classified into two groups: catalytic mutants with retained tetrameric structure but altered kinetic properties (W147 R, R303 W, and A337V) and structural mutations in which heterotetramers dissociate into subunits with impaired enzymatic activity (A149P, A174D, N334 K) [81]. Mutations upstream of the protein-coding region of ALDOB were first reported in an analysis of 61 patients with HFI that revealed single base mutations in the promoter, intronic enhancer, and first exon, which is entirely untranslated. These novel mutations represent 2% of alleles in American patients with HFI [82]. Pathogenic variants in aldolase B are kept in online databases such as www.bu.edu/aldolase/HFI/hfidb/hfidb.html and www.hgmd.cf.ac.uk/ac/gene.php?gene=ALDOB.
Clinical Presentation
Patients with HFI may be extremely ill and may die after continuous exposure to fructose. However, affected patients are generally healthy and symptom free so long as they do not ingest fructose or fructose-containing foods (including sucrose and sorbitol) [83]. For this reason, symptoms do not arise until breast milk or cow’s milk formulae are supplemented with fructose-containing foods. Nevertheless, some infant formulas do contain sucrose (e.g., Similac Sensitive – labeled as “sugar” [84]) and sucrose is often used in hospital as an analgesic in neonates undergoing painful procedures. In fortunate children, fructose is not introduced until after an affected infant is five to six months of age. By this time, the child is likely to associate nausea, vomiting, and symptoms of hypoglycemia with sweet-tasting food. In such cases, aversion to sweets is probably lifesaving, and the diagnosis may go undetected until adulthood. When this occurs, the diagnosis may be suspected on the basis of a careful history that recognizes the extreme aversion to dietary “sweets.” Symptoms associated with HFI may be categorized as resulting from acute or chronic exposure to fructose leading to the accumulation of fructose 1-phosphate in tissues in which aldolase B is normally present:
Acute
nausea, vomiting
tremor
dizziness
lethargy, coma
or chronic
failure to thrive
jaundice, cirrhosis, chronically increased transaminase levels
vomiting and diarrhea
feeding difficulties.
The largest single collection of patients consists of 55 patients diagnosed between 1961 and 1977 as having HFI [85]. Fifty had become symptomatic because of dietary fructose, and five were diagnosed shortly after birth because an older sibling of each infant was known to have HFI. Fourteen patients received fructose in their first feedings, and symptoms usually appeared within a few days. The remaining patients received a fructose-free diet (breast milk or cow’s milk formula). Their symptoms began immediately after introduction of dietary fructose or sucrose. Of the 50 patients, 32 (64%) were diagnosed as having HFI at less than six months of age, 12 (24%) between six and 12 months of age, and six (12%) after one year of age. The younger patients were usually admitted to the hospital on an emergency basis with acute liver impairment, sepsis, bleeding diathesis, shock, or dehydration. Patients younger than six months of age developed a triad of jaundice, edema, and bleeding tendency. Older patients were admitted more often because of liver enlargement, ascites, or both. Vomiting and hepatomegaly were observed in all patients, and about half had anorexia, weight retardation, and bleeding tendency. About a third had jaundice, diarrhea, edema or ascites or both, and growth retardation. An aversion to sweet foods was developed by 13 (26%), occurring as early as three months of age and in two children resulted in continued breast-feeding until nine months of age. Vomiting and diarrhea in the young children were sometimes severe enough to cause dehydration.
With greater awareness, more cases of HFI in children are being diagnosed, and the condition is arrested by feeding fructose-restricted diets. One cautionary note is that a number of proprietary milks, primarily the soy-based formulae, contain sucrose as a significant source of the carbohydrate calories. The remaining carbohydrate is usually a glucose oligosaccharide. Hypoglycemia and seizures may not be a problem in affected infants fed these formulae because the remaining carbohydrate is glucose. The liver disease caused by fructose ingestion may be progressive, however, and infants fed these formulae may simply fail to thrive, have hepatomegaly and vomiting, or progress to chronic liver failure and death. Acute liver failure in fructose intolerance is exceedingly unusual, and the absence of hepatomegaly in an infant who has severe liver disease and has reducing sugar in the urine should make one doubt the diagnosis of HFI. Follow-up studies of infants and recognition of older patients with HFI indicate a normal life expectancy. Patients retain their sensitivity to dietary fructose as adults, but the hypoglycemic response to fructose may be somewhat more delayed in adults than in infants (45–60 minutes in infants; 60–90 minutes in adults). The sensitivity to fructose may be life-threatening for adults. For example, patients with known HFI have been given sorbitol intravenously after surgery. Because sorbitol is metabolized to fructose, one patient died of complications from the sorbitol infusion [86].
Biochemical Features and Pathogenesis of Toxicity
Aldolase B is normally present in the liver, renal tubular cells, and intestinal mucosa [87]. It catalyzes the conversion of fructose 1-phosphate to D-glyceraldehyde and dihydroxyacetone phosphate (Figure 27.3). Fructose-1-P itself can be toxic by forming intracellular precipitates and can lead to necrosis of hepatocytes and renal tubular cells.
The metabolic consequences of this enzymatic deficiency are accumulation of large amounts of fructose 1-phosphate in the liver and depletion of inorganic phosphate (Pi) and ATP. While phosphorylation of fructose decreases intracellular phosphate also in normal individuals, the phosphate sequestered in normal liver is made available by further metabolism of fructose 1-phosphate. Therefore, changes in serum phosphate, secondary to the inability to release Pi from fructose 1-phosphate in cells of affected patients, are extremely transient in a normal individual and depend on the amount of fructose ingested.
One of the many effects secondary to this sequestration of Pi is an inability to regenerate ATP, a process that depends on the presence of Pi. The clinical and laboratory features of HFI can be understood on the basis of this simple scheme (Figure 27.4).
Accumulation of fructose 1-phosphate apparently causes the major manifestations of the disease through inhibition of other enzymatic reactions. Two metabolic pathways studied most extensively are gluconeogenesis and glycogenolysis. Their inhibition by fructose 1-phosphate explains fructose-induced hypoglycemia. Concentrations of fructose 1-phosphate in excess of 10 mmol/L completely inhibit fructose-1,6-biphosphatase activity in vitro [88]. This finding suggests that fructose 1-phosphate inhibits gluconeogenesis at this enzymatic step. Inhibition at this site is further supported by the finding that fructose-induced hypoglycemia is not prevented by simultaneous infusions of gluconeogenic precursors such as dihydroxyacetone or glycerol. In addition, liver specimens from patients with HFI do not form labeled glucose from [14C]-glycerol when fructose is present but oxidation of [14C]-glycerol is apparently unaffected by fructose [89].
Patients with HFI apparently also have inhibition of glycogenolysis after fructose intake. This inhibition occurs above the level of phosphoglucomutase. Probably a phosphate depletion causes an inhibition of glycogen phosphylase. Several studies of normal liver indicate that depletion of Pi as well as accumulation of fructose 1-phosphate may contribute to an almost complete failure of glycogen mobilization [90]. In addition, depletion of intracellular ATP levels may contribute to the lack of glycogen degradation to glucose 1-phosphate [91]. Also, glucagon does not increase blood glucose after fructose-induced hypoglycemia, even in the presence of normal to slightly elevated hepatic glycogen content [92].
Hypophosphatemia is likewise associated with hyperuricemia secondary to a depletion of ATP and Pi. This increases AMP deaminase activity leading to purine degradation to uric acid (Figure 27.4) [91]. Further ATP depletion leads to hypermagnesemia and to further liver and kidney toxicity.
Results of studies in newborn infants delivered at term suggest that they have less capacity for fructose metabolism in the first few days of life compared with later in life [93]. This is believed to be caused by the immaturity of the enzymes for handling fructose. In these studies, a rapid infusion of fructose caused a prompt but transient decrease in blood glucose and suppressed the glucagon-induced elevation of blood glucose. Although hepatic aldolase was not measured, the findings suggest that until further studies are completed, the use of fructose or sorbitol as a calorie source (as in total parenteral nutrition (TPN)) for term infants in the first few days of life may not be justified. Enzymatic maturation may take longer in premature infants, although definitive studies have not been reported. Intravenous preparations containing fructose/sucrose that once seemed appealing as they do not affect insulin production as much as glucose infusion, have been largely abandoned as this could lead to catastrophic presentation in these sometimes undiagnosed patients.
Laboratory Findings
The primary laboratory features of HFI are fructose-induced hypoglycemia and hypophosphatemia, hyperuricemia, fructosuria and/or chronic liver disease, as described above.
Elevation of serum levels of hepatic enzymes appears to be the direct effect of increased hepatic fructose 1-phosphate. Within 1.5 hours of a large dose of fructose, serum aminotransferase levels may increase more than two-fold. The mechanism of liver cell damage is not clear, but it may result from a combination of depletion of ATP and a direct toxic effect of elevated levels of the phosphorylated hexose.
Odievre and colleagues showed that the laboratory findings in HFI were actually variable [85]. Liver tests were severely deranged in the younger patients in their series. Deficiency of clotting factors and elevated alanine aminotransferase were present in all but one of the patients younger than six months of age. Two patients also had serum albumin of 2.8 g/dL. Fifteen patients had aminoaciduria; the predominant amino acids were tyrosine and methionine in three of these. All patients showed complete resolution of laboratory abnormalities in response to removal of fructose from the diet during a succeeding two-week period.
Other findings are less consistent. Some patients show substantial decreases in serum potassium and increases in serum magnesium after fructose intake. Some have increases in serum lactate and pyruvate [83, 94]. These changes appear to be related to the extent of liver damage and the severity of hypoglycemia. Granulocytosis may be noted with chronic fructose ingestion. As blood glucose declines after fructose ingestion, insulin and insulin-like activity decrease and levels of glucagon, epinephrine, and growth hormone increase. In response to these hormonal changes, the non-esterified fatty acids in plasma increase more than two-fold, a response not observed in normal subjects [83, 94, 95].
Renal tubular acidosis and a Fanconi-like syndrome with renal tubular re-absorptive defects have been reported [85]. In one patient, renal tubular acidosis persisted despite restriction of dietary fructose. The renal tubular acidosis is normalized in most patients as soon as fructose intake ceases [96]. Fructose 1-phosphate aldolase is normally present in the renal tubules but it is absent in patients with HFI. Hence, the transient renal disturbance in affected patients may be from accumulation of fructose 1-phosphate in renal tubular cells after fructose intake [87].
Diagnosis
Because the clinical presentation of HFI is highly variable and many of its characteristic features commonly occur with other disorders, the differential diagnosis may include hepatitis, intrauterine infection, septicemia, hemolytic uremic syndrome, galactosemia, tyrosinosis, Wilson disease, and other storage disorders. A detailed nutritional history correlating onset of symptoms with intake of fructose-containing foods is often a key component in the diagnostic process. Suspicion is fostered by the presence of reducing substances in urine. Although various conditions may be associated with hypoglycemia, most of them are associated with fasting. Hypoglycemia after eating should be a clue to the possibility of HFI. Other diseases that are associated with hypoglycemia following ingestion of food include deficiency of galactosemia and hyperinsulinism. Traditionally adults with undiagnosed aldolase B deficiency have been described to have a complete lack of dental carries due to self-restriction of sweets.
Of note, there is a distinction between hereditary fructose intolerance (aldolase B deficiency) and fructose malabsorption. The latter can also present with gastrointestinal symptoms (bloating, diarrhea, and abdominal discomfort), however, there is no hepatic involvement. Probably this is caused by malabsorption of fructose in the intestine (GLUT5-deficiency). The diagnosis of fructose malabsorption can be made by an oral fructose challenge test. This test should never be used in HFI as this could lead to severe liver disease and hypoglycemia as described above.
Historically, direct measure of fructose 1-phosphate aldolase in hepatic or small intestine tissue samples has been employed in the diagnosis of HFI. The liver is the preferred source for biopsy specimens because an assessment of tissue damage, as indicated by the presence of limited and scattered necrosis of hepatocytes, intralobular and periportal fibrosis, and diffuse fatty vacuolization resulting from fructose 1-phosphate accumulation, can be made simultaneously [85]. Assay of enzyme activity in serum and blood cells shows only slightly reduced levels and is of little diagnostic value. Currently, more than 95% of patients with HFI can be diagnosed through amplification of DNA with a limited number of allele-specific oligonucleotides, circumventing the need for tissue biopsy [78].
Treatment
A diet containing no fructose alleviates all the symptoms and liver dysfunction associated with HFI [78, 81, 83]. It is important that children and their parents receive detailed dietary counseling about which foods contain fructose. Older children commonly may associate discomfort with specific foods and regularly avoid them. However, infants are completely dependent on dietary selections made by their parents. Sorbitol also must be eliminated, because of its conversion to fructose in the human body. The common practice of adding small amounts of sugar to processed foods demands almost constant attention to avoid substantial fructose intake. Most of the sugars (for example fructose, high-fructose corn syrup, honey, agave syrup, inverted sugar, maple-flavored syrup, molasses, palm or coconut sugar, and sorghum) are not allowed. A complete list can be found on www.bu.edu/aldolase/HFI/treatment/index.html . Dietary guidance at an experienced metabolic center is required. In addition medicine and formulas can contain fructose, sucrose or sorbitol. This should always be checked by a pharmacist as package labels tend to be incomplete. Given the restriction on fruits and vegetables, supplements of a sugar-free vitamin are necessary [177].
Prognosis
Patients maintained on fructose-free diets have developed entirely normally, with normal lifespans, although most continue to have slight hepatomegaly with hepatic steatosis [85]. Even infants with severely deranged liver function and substantial hepatic fibrosis can achieve remarkable recoveries once fructose is removed from their diets.
Fructokinase Deficiency
This condition is also known as essential fructosuria. Due to the inability of fructokinase to phorphorylate fructose, fructose remains in the blood and eventually filters through the kidneys, hence causing fructose-uria. As glucokinase and hexokinase can also phosphorylate fructose, be it at a slower rate, this is essentially a non-disease. Except for the fructosuria, there are no other symptoms.
Fructose-1,6-Biphosphatase Deficiency
Fructose-1,6-biphosphatase (FBP) deficiency, although here described in the fructose metabolism section, is actually a defect in the gluconeogenesis and typically presents as a typical energy-type disorder metabolic disease with fasting-induced hypoglycemia with life-threatening lactic acidosis and liver dysfunction.
In 1970, Baker and Winegrad described the first patient with deficient hepatic FBP activity [67]. Other patients with similar clinical and laboratory findings have subsequently been reported [97]. Typically the patients present during the first days of life. The primary difference between patients with FBP deficiency and patients with HFI is that fasting as well as dietary fructose induces symptoms in these patients and there are no gastrointestinal symptoms. Several patients have been found to have “partial” FBP deficiencies. These patients do not have lactic acidosis but do develop hypoglycemia during fasting or secondary to dietary intake of fructose or glycerol.
Lab reports often show elevated triglycerides as they measure glycerol which is elevated as it is blocked in the gluconeogenesis. Likewise pyruvate and alanine are elevated. Furthermore the formed malonylCoA inhibits fatty acid oxidation.
Fructose-1,6-biphosphatase deficiency is inherited as an autosomal recessive trait. The diagnosis can be made by measurement of FBP in cultured lymphocytes and confirmed by detections of mutations in FBP1 (encoding FBP) [98].
Therapy in the acute life-threatening presentation consists, as in all energy disorders, of high dose glucose infusion (e.g., glucose 10% at 1.5 times normal maintenance supplemented with electrolytes as needed) and bicarbonate. Fasting should always be avoided. Dietary measures include the restriction of fructose and sucrose and the use of enteral feeding or uncooked cornstarch to provide continuous caloric support.
Glycogen Storage Diseases
Glycogen, a polysaccharide, is the primary carbohydrate storage compound in animals. It is present in virtually all animal cells and is particularly abundant in liver and muscle tissue. It undergoes de-polymerization through phosphorolysis and hydrolysis to release free glucose as needed to sustain cellular processes and to maintain normal blood glucose concentrations during fasting. The formation and degradation of glycogen are highly regulated processes involving many enzymes. Deficiencies of each enzyme have been identified in humans and result in one of the 15 recognized forms of GSD (Figure 27.5). In most types of GSD, the glycogen content of liver or muscle or both is excessive. In unusual cases, the glycogen content may be less than normal, the molecular structure of glycogen may be abnormal, or both may occur. Despite differences in the specific enzymatic defects, most of the syndromes are not readily distinguishable on clinical grounds alone, and tissue analyses for glycogen content, enzymatic activity or genetics are necessary to confirm the diagnoses. The discussion here is limited to types I (defect in glycogenosis), III (amylo-1,6-glucosidase deficiency), and IV (α-1,4 glucan-6-glycosyl transferase deficiency) because their clinical expressions primarily involve the liver; however, the clinical features of all GSDs are provided in Table 27.1 [99].
Figure 27.5 Pathway for glycogen synthesis and degradation to glucose. Broken lines indicate enzymatic activation after glucagon stimulation; heavy arrows indicate glycogen degradation from glucagon infusion; boxes across lines indicate points in the metabolic sequence where enzymatic defects have been identified.
Type | Gene (Enzyme) | Variants | Clinical Symptoms |
---|---|---|---|
I | GALT (Galactose-1-posphate-uridilyltransferase) | Classic | Life treathening: liver, long-term complications (neurology, cognitive, behavior, ovarian insufficiency, osteopenia) |
Clinical variant | Life-threatening, complications are preventable | ||
Biochemical variant (Duarte) | Not life-threatening (no treatment) | ||
II | GALK1 (galactokinase) | Cataracts | |
III | GALE (UDP-galactose-4-epimerase) | Systemic | Life-threatening: liver, long-term complications (neurology, cognitive, behavior, ovarian insufficiency, osteopenia) |
Peripheral (blood cells) | None | ||
IV | GALM (Galactose-mutarotase) | Cataracts | |
Other | Porto-systemic shunts, citrin deficiency and Fancon–Bickel syndrome |
Biochemistry of Glycogen Metabolism
Glycogen is a polymer of glucose units linked between the C-1 of one D-glucopyranosyl residue and the hydroxyl at C-4 of the adjacent residue (1,4-linkage). Short chains of glucose residues linked through the hydroxyl groups at C-6 of some of the residues (α-1,6-linkage) represent 7–8% of the glycogen, which allows a highly branched structure. The role of glycogen in the liver is to provide glucose to the blood for various organs. At times of stress or if blood glucose levels fall, typically a few hours after a meal, the liver rapidly releases glucose into the bloodstream, which carries it to organs such as the brain. Glycogen in the muscle serves as a reserve of glycolytic fuel to be used locally if oxygen or glucose availability declines.
Glycogen is synthesized from and degraded to glucose (Figure 27.5). Glycogen synthesis occurs through the action of glycogen synthase and branching enzymes. Hydrolysis occurs through phosphorylase and amylo-1,6-glucosidase. Glycogen synthase catalyzes the synthesis of glycogen from UDP-glucose. Several protein kinases can phosphorylate glycogen synthase. Branching of glycogen is carried out by the transfer of α-1,4-linked glucosyl units from the outer chains of glycogen into a 1,6-position. Glycogen phosphorylase is an interconvertible enzyme, with the α and β forms representing the active and inactive forms, respectively. The phosphorylase enzyme catalyzes the transfer of a glucose unit at the non-reducing end of α-1,4-glucosyl chain glycogen to liberate glucose 1-phosphate. Activation of this enzyme by epinephrine and glucagon plays a major role in controlling glycogenolysis. After extensive phosphorylase action on glycogen, the molecule contains four glucose residues in α-1,4-glucosidic bonds attached by an α-1,6-link. This unit is termed phosphorylase limit dextrin. The enzyme oligo-(1,4→1,4)-glucan transferase removes three of the four glucose residues, exposing the 1,6-linkages to be acted upon by the amylo-1,6-glucosidase enzyme to yield free glucose. Both enzymes represent the catalytic activity of the debrancher enzyme.
Because glycogen contains 8% branch points (1,6-links), glycogen degradation by phosphorylase and debrancher enzymes yields about 8% free glucose. The major end-product of glycogen hydrolysis by phosphorylase is glucose 1-phosphate, which is acted on by the enzyme phosphoglucomutase to yield glucose 6-phosphate. There is no known deficiency of the enzyme phosphoglucomutase. Glucose-6-phosphatase (G6Pase) is responsible for the formation of the majority of glucose from gluconeogenesis and glycogenolysis; it is a microsomal enzyme that catalyzes the hydrolysis of glucose 6-phosphate into glucose and phosphate. Glucose-6-phosphatase is present in the liver and kidney and in intestinal mucosa.
The control of glycogen metabolism is mediated by several factors that control glycogen synthesis and degradation by the enzymes glycogen synthetase and phosphorylase, respectively. Control of these enzymes occurs through several factors, including hormonally mediated changes in the concentrations of glucose and glycogen [100]. During feeding, high glucose concentration in the sinusoids allows glucose to bind to the phosphorylase enzyme and causes conversion of active phosphorylase into inactive phosphorylase, resulting in a halt in glycogenolysis. Because active phosphorylase is an inhibitor of the synthase, its inactivation allows glycogen synthesis to proceed. During fasting, a hormonally mediated (glucagon) increase in cAMP allows activation of protein kinase, which converts inactive to active phosphorylase, initiating glycogenolysis. High glycogen content also favors glycogenolysis by inhibiting glycogen synthase.
Table 27.2 Glycogen storage diseases affecting liver function
Number | Enzyme deficiency | Gene(s) | Main phenotype(s) |
---|---|---|---|
0a | Liver glycogen synthase | GYS2 | Hypoglycaemia Postprandial hyperglycemia Ketosis |
Ia | Glucose-6-phosphatase, or von Gierke disease | GSPC | Hypoglycaemia, Hepatomegaly Lactic acidosis |
Ib | Glucose-6-phosphate transport | SLC37A4 | Hypoglycaemia, Hepatomegaly Lactic acidosis Neutrophil dysfunction, Colitis |
III | Glycogen debrancher or Cori disease | AGL | Hypoglycaemia, Hepatomegaly |
IV | Glycogen brancher or Andersen disease | GBE1 | Cirrhosis; Myopathy/cardiomyopathy; Adult polyglucosan body disease |
VI | Liver glycogen phosphorylase | PYGL | Hepatomegaly, hypoglycaemia, growth delay |
IXa | Phosphorylase kinase (various subunits Table 5.2) | PHKA2 | Hepatomegaly, hypoglycaemia, growth delay |
IXb | PHKB | ||
IXc | PHKG2 | ||
XI | GLUT2 or Fanconi Bickel syndrome | SLC2A2 | Hepatomegaly, hypoglycaemia, rental tubular disease, growth delay |
Glycogen Storage Disease Type IA
In 1929, von Gierke published detailed reports of autopsies of two young children in which the most remarkable findings were excessive glycogen accumulation in hepatic and renal tissues, resulting in three-fold and two-fold increases, respectively, in organ size [101]. In the 1950s, further investigation by Cori and Cori showed that hepatic G6Pase activity was deficient in patients with von Gierke disease [102]. In 1954, von Gierke disease was classified as GSD-I [103]. It is the most commonly diagnosed form of hepatic glycogenosis, representing approximately 25% of all cases.
In GSD-I, there is a defect in conversion of glucose 6-phosphate to glucose as in normal glycogenolysis and gluconeogenesis. Currently two types of GSD-I are recognized: GSD-IA, a deficiency in G6Pase, and GSD-IB, a deficiency in glucose 6-phosphate transporter [104].
Molecular Basis
The cDNA encoding the murine G6Pase was cloned by screening a mouse liver cDNA library differentially with mRNA populations representing the normal and the albino deletion mouse known to express markedly reduced level of G6Pase [105]. This discovery allowed the cloning of the human G6Pase cDNA by homology screening. The human gene (G6PC) spans 12.5 kb, composed of five exons, and encodes for a protein of 357 amino acid residues [106]. The gene has been localized to chromosome 17q21. To date, more than 84 mutations have been identified in the gene in patients with GSD-IA [106, 107]. The two most common mutations give rise to R83C and Q347X, which account for more than 70% of mutations in Caucasian populations [109]. The common mutated form Q347X has a protein truncation of the last 10 C-terminal amino acids that contain the signal for retention of the enzyme in the endoplasmic reticulum. Sequence analysis of G6PC can diagnose up to 94% of the affected individuals [108].
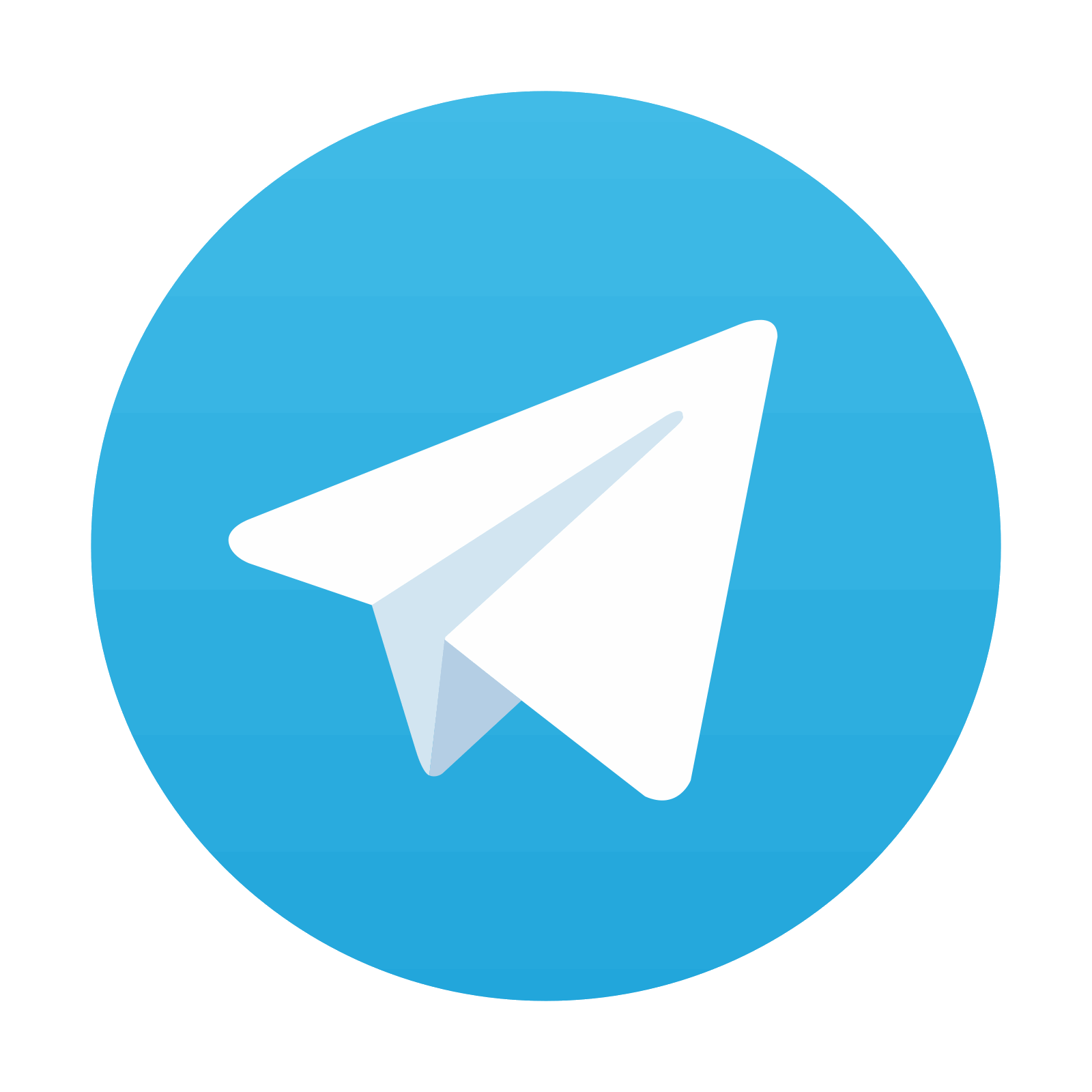
Stay updated, free articles. Join our Telegram channel
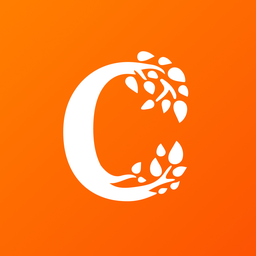
Full access? Get Clinical Tree
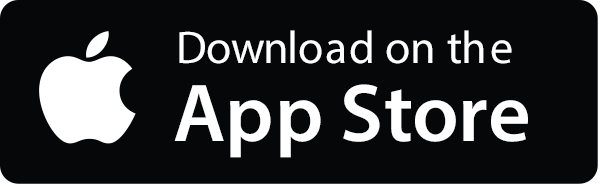
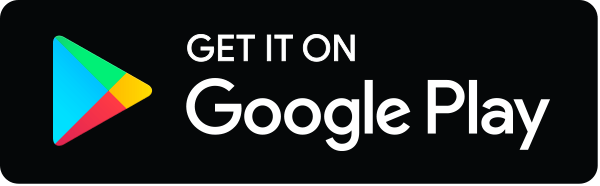
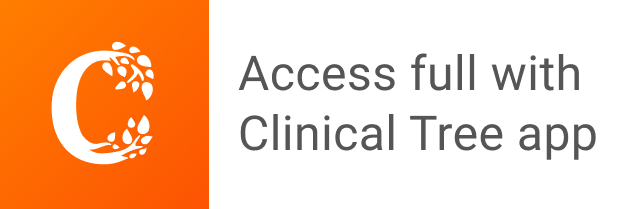