Abstract
Homozygous (PiZZ phenotype1) α1-antitrypsin (α1-AT) deficiency is a relatively common autosomal codominant genetic disorder, affecting 1 in 1,600–3,000 live births in most populations of Northern European ancestry [1, 2]. Although α1-AT deficiency liver disease develops in only a subset of PiZZ individuals, this condition represents the most common metabolic cause of liver disease and liver transplantation in children [3]. It can also cause chronic liver disease, hepatocellular carcinoma, and premature pulmonary emphysema in adults [3, 4].
Introduction
Homozygous (PiZZ phenotype1) α1-antitrypsin (α1-AT) deficiency is a relatively common autosomal codominant genetic disorder, affecting 1 in 1,600–3,000 live births in most populations of Northern European ancestry [1, 2]. Although α1-AT deficiency liver disease develops in only a subset of PiZZ individuals, this condition represents the most common metabolic cause of liver disease and liver transplantation in children [3]. It can also cause chronic liver disease, hepatocellular carcinoma, and premature pulmonary emphysema in adults [3, 4].
α1-Antitrypsin is an approximately 55 kDa secretory glycoprotein that inhibits destructive neutrophil proteases, elastase, cathepsin G, and proteinase 3. Plasma α1-AT is derived predominantly from the liver and increases three-to-five-fold during the host response to tissue injury or inflammation. It is the archetype of a family of structurally related circulating serine protease inhibitors called serpins. The deficient PiZZ state is associated with an 85–90% reduction in serum α1-AT concentrations. A single amino acid substitution results in an abnormally folded α1-AT protein, designated α1-ATZ, which is unable to traverse the secretory pathway. The mutant α1-ATZ protein is retained in the endoplasmic reticulum (ER) rather than secreted into the blood and body fluids. The PiZZ-deficient state is unique as a genetic disease in that it causes injury to one organ, the lung, by a loss-of-function mechanism, and injury to another organ, the liver, by a gain-of-hepatotoxic-function mechanism. This latter consideration has been best demonstrated in mice transgenic for mutant human α1-ATZ, in which ATZ accumulates in the liver [5, 6] and causes hepatic fibrosis [7] and carcinoma [8].
Although many α1-AT-deficient individuals develop destructive lung disease and emphysema as adults, that condition does not affect children. Most data in the literature indicate that emphysema results from a mechanism whereby decreased numbers of α1-AT molecules within the lower respiratory tract allow unregulated elastolytic attack on the connective tissue matrix of the lung [4]. Oxidative inactivation of residual α1-AT as a result of cigarette smoking accelerates lung injury. Moreover, the elastase–antielastase theory for the pathogenesis of emphysema is based on the concept that oxidative inactivation of α1-AT as a result of cigarette smoking plays a key role in the emphysema of α1-AT-sufficient individuals, the vast majority of patients with emphysema [4].
Although it is a single gene defect, there is extraordinary variation in the phenotypic expression of liver disease in the classical PiZZ form of α1-AT deficiency. A nationwide prospective screening study by Sveger and co-workers in Sweden showed that only 22 infants from an unbiased cohort of 127 PiZZ subjects developed prolonged obstructive jaundice or other reasons for suspicion of clinically significant neonatal liver disease [1]. These data indicate that other genetic traits and/or environmental factors predispose a subgroup of PiZZ individuals to liver disease. With respect to lung disease in adults with α1-AT deficiency, environmental factors such as cigarette smoking obviously play an important role in the phenotypic expression [9]. Interestingly, studies show that other genetic traits also influence the manifestation incidence and severity of lung disease [10].
Historically, the diagnosis of α1-AT deficiency was based on the altered migration of the abnormal α1-ATZ molecule in serum specimens subjected to isoelectric focusing gel analysis. Contemporary diagnostic strategies may now employ genomic analyses for liver disease-associated α1-AT gene mutations. Treatment of α1-AT deficiency-associated liver disease is mostly supportive. Liver transplantation has been used successfully for severe liver injury. α1-AT-deficient patients with severe emphysema have undergone lung transplantation. Augmentation therapy with intravenous administration of purified plasma α1-AT was approved for α1-AT deficiency lung disease on the basis of biochemical efficacy studies [11]. A recent randomized, placebo-controlled trial provided some evidence that augmentation therapy slows the progression of lung destruction in α1-AT deficient subjects [12]. Nevertheless, this strategy has not obviated the need for lung transplantation in some patients with emphysema due to α1-AT deficiency. Several new pharmacologic and genetic strategies for prophylaxis of both liver and lung disease are under development for clinical application.
Recent studies of other genetic diseases show that α1-AT deficiency liver disease is a prototype for diseases in which mutant toxic proteins accumulate in an inappropriate subcellular compartment. Basic cell biology studies of protein folding and trafficking have also identified conserved protein homeostasis (i.e., proteostasis) pathways within cells that serve as protective mechanisms against the protein-induced toxicity (i.e., proteotoxicity) associated with inappropriate accumulation of mutant proteins in various specific organelles. These “quality control” processes are mediated by molecular chaperones, folding catalysts, and translocation processes by which mutant proteins can be delivered to degradative systems or aggregated and sequestered within the cell so as to limit potential damage. There are also now well-described protective signal transduction pathways, such as the unfolded protein response (UPR), through which the cell can produce more quality control components to prevent such damage. Better understanding of these mechanisms of protein homeostasis (proteostasis) has already resulted in several new genetic and pharmacological approaches to the prevention of liver and lung disease that are in various stages of development [13].
Clinical Manifestations
Liver Disease
Liver involvement is often first noticed in early infancy because of persistent jaundice (Table 25.1). Conjugated bilirubin and serum aminotransferase levels in the blood are mildly-to-moderately elevated. Blood levels of alkaline phosphatase and gamma-glutamyltransferase also may be elevated. The liver may be enlarged. There is a tendency for some affected infants to be small for gestational age. Because these clinical and laboratory characteristics are similar to those that occur in other causes of liver injury in the newborn period, these infants may initially be given the diagnosis of neonatal hepatitis syndrome and subjected to a diagnostic evaluation for various disorders including α1-AT deficiency [14]. Infants also may be evaluated initially for α1-AT deficiency because of an episode of gastrointestinal bleeding, bleeding from the umbilical stump, or bruising. A small number of affected infants, approximately 10% of the deficient population, have hepato-splenomegaly, ascites, and liver synthetic dysfunction in early infancy. An even smaller number have severe fulminant liver failure in infancy [14]. A few infants are recognized initially because of a cholestatic clinical syndrome characterized by pruritus and hypercholesterolemia. The clinical picture in these patients resembles extrahepatic biliary atresia, but histologic examination shows paucity rather than proliferative expansion of intrahepatic bile ducts.
Liver disease associated with α1-AT deficiency may also first be discovered in late childhood or early adolescence, when the affected individual develops abdominal distension from hepatosplenomegaly or ascites, has splenomegaly, or has upper intestinal bleeding caused by esophageal variceal hemorrhage. In some of these patients, there is a history of unexplained prolonged obstructive jaundice during the neonatal period. In others, there is no evidence of any previous liver injury, even if the neonatal history is carefully reviewed (Table 25.1).
Table 25.1 Clinical and Diagnostic Features of Liver Disease Associated with α1-Antitrypsin Deficiency
Clinical features | Infants | Prolonged jaundice |
Neonatal hepatitis syndrome | ||
Toddler | Mild elevation of aminotransferases | |
Child/Adolescent | Portal hypertension | |
Severe liver dysfunction | ||
Adult | Chronic hepatitis | |
Cryptogenic cirrhosis | ||
Hepatocellular carcinoma | ||
Diagnostic features |
|
Deficiency of α1-AT should be considered in the differential diagnosis of any adult who presents with chronic hepatitis, cirrhosis, portal hypertension, or hepatocellular carcinoma of unknown origin. An autopsy study in Sweden showed a higher risk of cirrhosis in adults with α1-AT deficiency than was previously suspected and that α1-AT deficiency has a strong association with primary liver cancer [15]. Moreover, the risk of liver cancer was greater than could be accounted for by the known increase associated with cirrhosis alone [15]. Interestingly, cirrhosis and liver cancer may be initially diagnosed in a patient with little in the way of clinical manifestations of liver disease, perhaps only asymptomatic hepatomegaly or elevated aminotransferases or bilirubin levels. Primary liver cancer is also observed in the absence of cirrhosis in some patients with α1-AT deficiency [16]. The histology of the hepatic cancer can be characteristic of hepatocellular carcinoma or cholangiocarcinoma, or have features of both [16]. Although it has been said that heterozygotes are at increased risk of primary liver cancer, it has not been possible to prove that the cancers in these patients are caused by the allelic variant itself.
The only prospective data on the incidence and natural history of α1-AT deficiency-associated liver injury is the Swedish nationwide screening study done by Sveger [1]. In this study, 200,000 newborn infants were screened and 127 PiZZ individuals identified and prospectively followed, most recently restudied at age 37–40 years [17]. The initial analysis of this cohort identified 14 PiZZ infants with prolonged cholestatic jaundice, another eight with other clinical- or laboratory-based suspicions for liver disease, and the remainder without any evidence of liver disease. Published follow-up studies of the original cohort reported that five PiZZ subjects subsequently died in childhood, two with known cirrhosis and two others with liver disease incidentally discovered at autopsy [18]. At the most recent reassessment of this cohort, at age 37–40 years, no participating subjects showed evidence of active liver disease [17]. However, abnormalities in liver-related imaging and/or laboratory studies were reported. One issue not yet addressed by Sveger’s or any other study is whether some PiZZ subjects have subclinical histologic abnormalities that eventually manifest as clinically evident liver disease in later life.
It has also become evident that α1-AT deficiency can cause liver disease with initial onset in adulthood. Our recent retrospective analysis of US liver transplantation databases found that 77% of α1-AT-deficient subjects undergoing liver transplantation over the last 20 years were adults, with peak age of transplantation in those subjects of 50–64 years [19]. Although many of these subjects were probably heterozygous for the ATZ gene mutation with other potential causes of liver disease, and some diagnoses may have been poorly substantiated, our analysis nevertheless suggests that severe liver disease attributed to α1-AT deficiency and requiring transplantation in subjects with PiZZ or PiSZ2 phenotypes is more frequent in adults than children. This relatively new recognition that α1-AT deficiency-associated liver disease is prevalent in adults is consistent with the discovery that autophagy, which is a central mechanism of proteostasis, plays a critical role in the pathobiology of this liver disease. Furthermore, it is consistent with the understanding that what appears to be a physiological decline in autophagic function in middle age may play an important role in age-dependent degenerative diseases in a general way.
It remains unclear what clinical manifestations or abnormal laboratory test results can be used to predict a poor prognosis for individuals with α1-AT deficiency-associated liver disease. Even children with cirrhosis and portal hypertension can lead relatively healthy lives for years without the need for liver transplantation, or for years before the transplant procedure is needed [20]. Thus, the timing of liver transplantation should depend more on overall life functioning rather than any single clinical or biochemical determination.
Whether liver injury results from the heterozygous (i.e., PiMZ3) genotype of α1-AT by itself is also still uncertain. Studies of this issue are generally biased in ascertainment because the index population is derived from a pathologic registry or some other type of clinical database. Furthermore, existing studies do not include concurrent prospective controls [3]. Nevertheless, because of clinical experiences over many years in which no other explanation for severe liver disease in PiMZ heterozygotes was identified despite exhaustive diagnostic evaluation, we suspect that the lack of evidence for predisposition to liver disease in heterozygotes is because of difficulties in study design more than anything else. Ultimately, prospective longitudinal study of an MZ cohort, analogous to the Sveger study [1], would best inform this consideration.
Interestingly, recent studies have reported significant correlations between the MZ state and development of, presentation with, or more rapid progression to advanced liver disease and liver transplantation in subjects with other forms of chronic liver diseases [19, 21].These data have added additional circumstantial evidence to the concept that other chronic liver diseases, including viral hepatitis, fatty liver disease, and others, might modify the degree of hepatotoxicity caused by α1-ATZ expression in MZ subjects. They also emphasize the importance of excluding other causes when evaluating PiMZ patients with liver disease.
Liver disease has also been associated with several other allelic variants of α1-AT. For example, children with compound heterozygosity type PiSZ are affected by liver injury in a manner similar to PiZZ children [1, 22]. There are several reports of liver disease in the α1-AT deficiency variant PiMmalton. These are particularly interesting associations because the mutant PiMmalton and PiSZ α1-AT molecules have been shown to undergo polymerization and retention within the ER [3]. Liver disease has been detected in single patients with several other α1-AT allelic variants [3], but in those cases other causes of liver injury have generally not been completely excluded.
Historically, the diagnosis of α1-AT liver disease was established by a serum α1-AT phenotype determination using isoelectric focusing or agarose electrophoresis at acid pH. Modern genomic methodologies permit specific analyses for known liver disease-associated α1-AT gene mutations, and analyses for gene duplications, deletions, or previously unidentified mutations in the AT coding region. Based on considerations mentioned above related to the uncertainty about the role of heterozygous ATZ expression to liver injury in PiMZ subjects, when evaluating PiMZ patients with liver disease it is always important to exclude other causes.
Liver histology is characterized by periodic acid-Schiff positive, diastase resistant globules in the hepatocyte ER. These globules are most prominent in periportal hepatocytes, and may also be seen in Kupffer cells and cells of biliary ductular lineage (reviewed in [3, 14]). There may be variable hepatocellular necrosis, inflammatory cell infiltration, periportal fibrosis, and/or cirrhosis. There is often evidence of bile duct epithelial cell destruction, and occasionally paucity of intrahepatic bile ducts. There can also be an intense autophagic reaction with nascent and degradative type autophagic vacuoles detected by electron microscopy on liver biopsies (Figure 25.1, [23]).
Figure 25.1 Liver histology in homozygous PiZZ AT deficiency. (A) Micrograph of liver biopsy specimen demonstrating increased fibrous tissue deposition (arrowheads) and nodular regeneration. Periodic acid-Schiff (PAS)-diastase staining, 4x magnification. (B) Micrograph of liver biopsy demonstrating PAS-positive, diastase-resistant globules. (Two hepatocytes with globules are indicated by arrows, 40x magnification.) (C) Electron micrograph of same biopsy demonstrating globules (g) in ER. (n: nucleus; arrowheads identify ribosomes.)
Lung Disease
The incidence and prevalence of emphysema in α1-AT deficiency have not been studied prospectively. Autopsy studies suggest that 60–65% of people with homozygous PiZZ α1-AT deficiency develop clinically significant lung injury [2, 4]. There are smokers with α1-AT deficiency however, who do not have any symptoms of lung disease or evidence of pulmonary function abnormalities until the seventh to eighth decade of life [2]. The typical person with lung disease is a male cigarette smoker. Onset of dyspnea is insidious in the third to fourth decade of life. About 50% of affected people develop cough and recurrent lung infections. The disease progresses to a severe limitation of airflow. A reduction in the forced expiratory volume, an increase in total lung capacity, and a reduction in diffusing capacity occur. Chest radiographs demonstrate hyperinflation with marked lucency at the lung bases. Histopathologic studies demonstrate panacinar emphysema, more prominent in the lower lung [2, 4].
It is rare for emphysema to affect an α1-AT-deficient patient during childhood. A number of patients have been described in the literature, but for each of these, an alternative explanation can be offered [3]. A number of infants with α1-AT deficiency have had pulmonary function testing suggesting a subtle degree of hyperinflation. Another study, however, did not detect any significant difference between the pulmonary function of PiZZ children between the ages of 13–17 and that of an age-matched control group [3]. These data indicate that it is extremely rare for α1-AT deficiency to cause emphysema in individuals aged younger than 25 years.
The destructive effect of cigarette smoking on the outcome of lung disease in α1-AT deficiency has been demonstrated in many studies. Actuarial studies suggest that cigarette smoking reduces median survival by more than 20 years in deficient people. The rate of decline in forced expiratory volume is four-fold greater in smoking than non-smoking people with α1-AT deficiency [2, 4].
There is still limited information about the incidence of liver disease in α1-AT-deficient individuals with emphysema. In one study of 22 patients with PiZZ and emphysema, there were elevated serum aminotransferases in ten patients and cholestasis in one patient [24]. Although liver biopsies were not done in that study, a more recent comparative autopsy analysis of 41 PiZZ subjects enrolled in the Registry of Individuals with Severe Deficiency of AT also reported a lack of correlation between liver and lung pathology [25].
Physiology
Structure of α1-Antitrypsin
α1-Antitrypsin is encoded by a 12.2 kb gene (SERPINA1, Figure 25.2) containing seven exons and six introns on human chromosome 14q31-32.3 [3]. The first three exons and a short 5′ segment of the fourth encode variable, tissue-specific 5′ untranslated regions (UTRs) of the α1-AT mRNA, which are generated by alternative posttranscriptional exon splicing [3]. Such alternative splicing results in variable inclusion or exclusion of long upstream open reading frames whose presence or absence alters the efficiency of α1-AT translation [26] and could thus potentially affect the hepatic phenotype of PiZZ subjects. Most of the fourth exon and the remaining three exons encode the α1-AT protein sequence. The α1-AT protein is a single-chain, 55 kDa polypeptide with 394 amino acid residues and three asparagine-linked complex carbohydrate side-chains. The two major serum isoforms differ in the configuration of the carbohydrate side-chains.
α1-Antitrypsin is the archetype of the serpin (serine protease inhibitor) family [14]. Most serpins function as suicide inhibitors of specific target proteases. Others are not inhibitory, but rather form complexes with but do not inactivate their hormone ligands. A comparison of α1-AT with other members of the serpin supergene family has generated several important concepts about serpin structure and function. For instance, the reactive site “P1” residue of α1-AT and other serpins is the most important determinant of functional specificity for each molecule. This concept was dramatically confirmed by the discovery of α1-AT Pittsburgh, a variant in which the P1 residue of α1-AT, Met 358, is replaced by Arg. In this variant, α1-AT functions as a thrombin inhibitor, and severe bleeding diathesis results [27]. α1-AT is an inhibitor of serine proteases in general, but its physiological targets are probably only neutrophil elastase, cathepsin G and proteinase 3, proteases released by activated neutrophils, because the kinetics of association with these enzymes are dramatically more favorable than with other serine protease [28]. The C-terminal fragment of α1-AT and the other serpins also bears important structural and functional homology. A small fragment at this terminus is cleaved during formation of the inhibitory complex with serine protease. This C-terminal fragment possesses chemotactic activity. Moreover, this fragment bears the receptor-binding domain for cell surface binding, internalization of α1-AT–elastase and other serpin–enzyme complexes, and activation of a signal-transduction pathway for upregulation of α1-AT gene expression [14].
Structural variants of α1-AT in humans are classified according to the protease inhibitor (Pi) phenotype system as defined by agarose electrophoresis or isoelectric focusing of plasma [3]. The Pi classification assigns a letter to variants according to position of migration of α1-AT in these gel systems, using alphabetic order from low to high isoelectric point. For example, the most common normal variant migrates to an intermediate isoelectric point, designated M. People with the most common severe deficiency have an α1-AT allelic variant that migrates to a high isoelectric point, designated Z. More than 100 allelic variants of α1-AT have been reported [4]. Structural variants of α1-AT not associated with changes in serum concentration or functional activity from the normal range are termed normal allelic variants and include the M1, M2, M3, M1 (Ala 213), X, Christchurch, and PSaint Alban’s alleles [4]. In each case, a single relatively conservative substitution is present. The α1-AT variants in which α1-AT is not detectable in serum are called null allelic variants and, if inherited with another null- (or deficiency-)variant, are associated with premature emphysema [4]. Several types of defect, including insertions and deletions, appear to be responsible for these variants. In two instances, α1-AT NullIsola di Procida and α1-AT NullReidenburg, there is deletion of all α1-AT coding regions. In two other cases, α1-AT NullBellingham and α1-AT NullGranite Falls, α1-AT mRNA is undetectable. Three other null alleles result in truncated proteins that are degraded in the ER: NullMattawa, NullHong Kong, and NullClayton [3].
Several variants of α1-AT associated with a reduction in serum concentrations of α1-AT have been described and are called deficiency variants (Table 25.2). Some of these variants are not associated with clinical disease, such as the S variant [4]. Other deficiency variants are associated with emphysema [3, 4] such as MHeerlen, MProcida, MMalton, MDuarte, MMineral Springs, PLowell, and WBethesda. In two people with MMalton and one with MDuarte, hepatocyte α1-AT inclusions and liver disease have been reported [3]. In one person with the deficiency variant Sliyama, emphysema and hepatocyte inclusions were reported, but this person did not have liver disease [3]. Dysfunctional variants of α1-AT include α1-AT Pittsburgh [27]. There also is a decrease in serum concentration and functional activity for α1-AT MMineral Springs [3]. For several variants that have been identified in compound heterozygotes such as α1-AT F, α1-AT Null (Newport), and α1-AT Z Wrexham, it is unclear whether the variants result in normal, null, deficient, or dysfunctional changes [3].
Variant | Defect | Site | Clinical Disease | Cellular defect | |
---|---|---|---|---|---|
Liver | Lung | ||||
Z | Single base substitution M1 [Ala213] | Glu342-Lys | + | + | IC accumulation |
S | Single base substitution | Glu264-Val | − | − | IC accumulation |
MHeerlen | Single base substitution | Pro369-Leu | − | + | IC accumulation |
MProcida | Single base substitution | Leu41-Pro | − | + | IC accumulation |
MMalton | Single base deletion | Phe52 | + | + | IC accumulation |
MDuarte | Unknown | Unknown | +? | + | Unknown |
MMineral Springs | Single base substitution | Gly57-Glu | − | + | No function; EC degradation? |
Siiyama | Single base substitution | Ser53-Phe | − | + | IC accumulation |
PDuarte | Two base substitution |
| +? | + | Unknown |
PLowell | Single base substitution | Asp256-Val | − | + | IC degradation? |
WBethesda | Single base substitution | Ala336-Thre | − | + | Accelerated catabolism? |
ZWrexham | Single base substitution | Ser19-Leu | ? | ? | Unknown |
F | Single base substitution | Arg223-Cys | − | − | Unknown |
T | Single base substitution | Glu264-Val | − | − | Unknown |
I | Single base substitution | Arg39-Cys | − | − | IC degradation |
MPalermo | Single base deletion | Phe51 | − | − | Unknown |
MNichinan | Single base deletion and single base substitution |
| − | − | Unknown |
ZAusburg | Single base substitution | Glu342-Lys | − | − | Unknown |
King’s | Single base substitution | His334-Asp | + | IC accumulation | |
Mpisa | Single base substitution | Lys259-Ile | − | +? | IC accumulation |
Etaurisano | Single base substitution | Lys368-Glu | − | + | IC accumulation |
Yorzinuovi | Single base substitution | Pro391-His | +? | – | IC accumulation |
PiSDonosti | S variant defect + Single base substitution | S variant site + Ser14-Phe | − | +? | IC polymerization, degradation; reduced secretion |
PiTijarafe | Single base substitution | Ile50-Asn | − | − | IC polymerization, stabilization; reduced secretion |
PiSevilla | Single base substitution | Ala58-Asp | − | +? | IC polymerization, stabilization; reduced secretion |
PiCadiz | Single base substitution | Glu151-Lys | − | +? | None |
PiTarragona | Single base substitution | Phe227-Cys | − | +? | IC polymerization, degradation; reduced secretion |
PiPuerto Real | Single base substitution | Thr249-Ala | − | +? | IC polymerization, stabilization; altered glycosylation |
PiValencia | Single base substitution | Lys328-Glu | − | − | IC stabilization (reduced enzyme activity) |
Function of α1-Antitrypsin
α1-Antitrypsin is an inhibitor of serine proteases in general, but its most important targets are neutrophil elastase, cathepsin G, and proteinase 3: proteases released by activated neutrophils. Several lines of evidence suggest that inhibition of these neutrophil proteases is the major physiologic function of α1-AT [4]. First, individuals with α1-AT deficiency are susceptible to premature development of emphysema, a lesion that can be induced in experimental animals by instillation of excessive amounts of neutrophil elastase [29], chronic cigarette smoke exposure [30], and most recently by genetic disruption of all five mouse Serpina1 paralogs [31]. These observations have led to the concept that destructive lung disease may result from perturbations of the net balance of elastase and α1-AT within the local environment of the lung. Second, the kinetics of association for α1-AT and neutrophil elastase are more favorable, by several orders of magnitude, than those for α1-AT and any other serine protease [28]. Third, α1-AT constitutes more than 90% of the neutrophil elastase inhibitory activity in the one body fluid that has been examined, pulmonary alveolar lavage fluid.
α1-Antitrypsin acts competitively by allowing its target enzymes to bind directly to the P1 Met residue within its reactive center loop [14]. The reaction between enzyme and inhibitor is essentially second order, and the resulting complex contains one molecule of each of the reactants. A reactive-site peptide bond within the inhibitor is hydrolyzed during formation of the enzyme–inhibitor complex, however, hydrolysis does not proceed to completion. Rather, an equilibrium, near unity, is established between complexes in which the α1-AT reactive-site peptide bond is intact (native inhibitor) and those in which this peptide bond is cleaved (modified inhibitor). The complex of α1-AT and serine protease is a covalently stabilized structure that is resistant to dissociation by denaturing compounds, including sodium dodecyl sulfate and urea. The interaction between α1-AT and serine protease is “suicidal” in that the modified inhibitor is no longer able to bind with or inactivate the enzyme.
The net functional activity of α1-AT in complex biologic fluids may be modified by several factors [4, 14]. First, the reactive-site methionine may be oxidized and thereby rendered inactive as an elastase inhibitor. In vitro, α1-AT is oxidatively inactivated by activated neutrophils and by oxidants released by alveolar macrophages of cigarette smokers. Second, the functional activity of α1-AT may be modified by proteolytic inactivation. Several metallo- and thiol proteases can cleave and inactivate α1-AT.
Although α1-AT from the plasma or liver of individuals with PiZZ α1-AT deficiency is functionally active [4, 14], there may be a decrease in its specific elastase inhibitory capacity. Ogushi et al. have shown that the kinetics of association and the stability of complexes with neutrophil elastase were decreased significantly for α1-AT isolated from PiZZ plasma. There was no decrease in the functional activity of α1-AT from PiSS individuals (reviewed in [3]).
α1-Antitrypsin has also been shown to protect experimental animals from the lethal effects of tumor necrosis factor. Most of the evidence from these studies indicates that this protective effect results from inhibition of the synthesis and release of platelet-activating factor from neutrophils, presumably through the inhibition of neutrophil-derived proteases (reviewed in [3]).
Several studies indicate that α1-AT has functional activities other than inhibition of serine protease. The C-terminal fragment of α1-AT, which can be generated during the formation of a complex with serine protease or during proteolytic inactivation by thiol- or metalloproteases, is a potent neutrophil chemoattractant [14]. Furthermore, it has been shown to prevent cellular entry of HIV [32, 33].
Although effects on lymphocyte activities and immune and inflammatory function have been attributed to α1-AT [34], there are inherent conflicts in some of the reports, and the data have not been duplicated. There is no evidence that the immune response is altered systematically in α1-AT-deficient individuals.
Biosynthesis of α1-Antitrypsin
The predominant site of synthesis of plasma α1-AT is the liver. This is most clearly shown by conversion of plasma α1-AT to the donor phenotype after orthotopic liver transplantation [14]. It is synthesized in human hepatoma cells as a 52 kDa precursor, then undergoes post-translational, dolichol phosphate-linked glycosylation at three asparagine residues and tyrosine sulfation. It is secreted as a 55 kDa native single-chain glycoprotein with a half-time for secretion of 35–40 minutes [14].
Tissue-specific expression of α1-AT in human hepatoma cells is directed by structural elements within a 750-nucleotide region upstream of the hepatocyte transcriptional start site in exon Ic. Within these regions are structural elements that are recognized by hepatocyte nuclear transcription factors (HNFs) and CCAAT/enhancer binding protein (C/EBP) (Figure 25.2, [14]). HNF1α and HNF4 appear to be particularly important for expression of human SERPINA1 (encoding α1-AT). Two distinct regions within the proximal element bind these two transcription factors. In fact, substitution of five nucleotides within the region of nucleotides (–77 through –72) disrupts binding of HNF1α and dramatically reduces production of the human α1-AT in the liver of transgenic mice [14]. Substitution of four nucleotides at positions –118 through –115 disrupts the binding of HNF4 but does not alter expression of the human gene for α1-AT in the liver of adult transgenic mice. The latter mutation does result in a reduction in the production of human α1-AT in the liver during embryonic development. HNF1α and HNF4 have a synergistic effect on expression of the gene for α1-AT in hepatocytes and enterocytes [3].
Plasma concentrations of α1-AT increase three-to-five-fold during the host response to inflammation or tissue injury [14]. Because the source of this additional α1-AT has been thought to be the liver, α1-AT is known as a positive hepatic acute phase reactant. Synthesis of α1-AT in human hepatoma cells (HepG2, Hep3B) is upregulated by interleukin-6 but not by interleukin-1 or tumor necrosis factor. Plasma concentrations of α1-AT also increase during oral contraceptive therapy and pregnancy [14].
α1-Antitrypsin is also synthesized and secreted in primary cultures of human blood monocytes and bronchoalveolar and breast milk macrophages [35]. Expression of α1-AT in monocytes and macrophages is influenced by products generated during inflammation, such as bacterial lipopolysaccharide and interleukin-6 [14].
Production of α1-AT is also regulated by a feed-forward mechanism in which elastase–α1-AT complexes mediate an increase in synthesis of α1-AT through the interaction of a pentapeptide domain in the C-terminal tail of α1-AT with a novel cell surface receptor [14]. This class of receptor molecules is now referred to as serpin–enzyme complex (SEC) receptors because they recognize the highly conserved domains of other SECs, such as antithrombin III–thrombin, α1-antichymotrypsin–cathepsin G, and, to a lesser extent, C1 inhibitor–C1s and tissue plasminogen activator–plasminogen activator inhibitor 1 complexes, as well as that of elastase–α1-AT complexes [14]. Substance P, several other tachykinins, bombesin, and the amyloid-β peptide bind to the SEC receptor through a similar pentapeptide sequence [14]. The SEC receptor can mediate endocytosis of soluble amyloid-β peptide, but it does not recognize the aggregated form of amyloid-β peptide, which is toxic to neurons and other cell types [3]. The SEC receptor may play a role in preventing amyloid-β peptide from accumulating in the amyloid deposits associated with Alzheimer disease.
α1-Antitrypsin mRNA has been isolated from multiple tissues in transgenic mice [36, 37], but in many cases it has not been possible to distinguish whether this mRNA is in ubiquitous tissue macrophages or other cell types. α1-AT is synthesized in enterocytes and Paneth cells, as indicated by studies in intestinal epithelial cell lines, ribonuclease protection assays of human intestinal RNA, and in situ hybridization analyses in cryostat sections of human intestinal mucosa [3]. Production of α1-AT in enterocytes increases during differentiation from crypt to villus, in response to interleukin-6 and during inflammation in vivo. It is also synthesized by pulmonary epithelial cells [3]. Interestingly, synthesis of α1-AT in pulmonary epithelial cells is less responsive to regulation by interleukin-6 than by a related cytokine, oncostatin M [12].
Clearance and Distribution of α1-Antitrypsin
The half-life of α1-AT in plasma is approximately five days [4]. It is estimated that the daily production rate of α1-AT is 34 mg/kg body weight, with 33% of the intravascular pool of α1-AT degraded daily. Several physiological factors may affect α1-AT catabolism, including its desialylation, proteolytic modification, and complex formation with elastase. The SEC and low-density lipoprotein receptor-related protein (LRP) receptors may also be involved in α1-AT clearance and catabolism (reviewed in [3, 14]). There is a slight increase in the rate of clearance of radiolabeled α1-ATZ compared with wild-type α1-AT if infused into PiMM individuals, but this difference does not account for the decrease in serum levels of α1-AT in deficient individuals [4].
α1-Antitrypsin diffuses into most tissues and is found in most body fluids [4]. Its concentration in lavage fluid from the lower respiratory tract is approximately equivalent to that in serum; it is also found in feces, and increased fecal concentrations of α1-AT correlate with the presence of inflammatory lesions of the bowel [3]. In each case, it has been assumed that α1-AT was derived from serum. Local sites of synthesis, however, such as macrophages and epithelial cells, also may make important contributions to the α1-AT pool in these tissues and body fluids. It has been reported that the rate of fecal α1-AT clearance is higher in patients with homozygous PiZZ α1-AT deficiency than in normal people [3]. Because the former have only 10–15% of the normal serum concentrations of α1-AT, a local intestinal source for fecal α1-AT is implicated. One possible explanation is that the bulk of α1-AT in feces is derived from sloughed enterocytes. Increased fecal α1-AT in those with homozygous PiZZ α1-AT deficiency would result from intracellular accumulation of the abnormal α1-AT molecule in enterocytes that are being sloughed at the usual rate. Increased fecal α1-AT in normal PiMM people with inflammatory-related, protein-losing enteropathy would result from increased sloughing of enterocytes alone.
Pathophysiology
Mechanism for Deficiency of α1-Antitrypsin in PiZZ individuals
The mutant α1-ATZ molecule is characterized by a single nucleotide substitution that results in an amino acid substitution, Lys for Glu 342 [14]. There is a selective decrease in the secretion of α1-AT, with the mutant protein accumulating in the ER [14]. The defect is not specific for liver cells; it also affects extrahepatic sites of α1-AT synthesis, such as macrophages and transfected cell lines [14]. Site-directed mutagenesis studies have shown that this single amino acid substitution is sufficient to produce the cellular defect [14]. Once translocated into the lumen of the ER, the mutant α1-AT protein is unable to traverse the remainder of the secretory pathway because it is folded abnormally.
Studies by Carrell, Lomas, and colleagues were the first to show that the human α1-ATZ protein is prone to polymerization and aggregation in liver and plasma [38–40]. These authors proposed a mechanism for ATZ polymerization that involved a unique “loop-sheet insertion” phenomenon. Polymers have also been found in the plasma of patients with the PISiiyama and PIMMalton α1-AT variants [41, 42], and those mutations (Table 25.2) are also expected to be susceptible to loop-sheet polymerization [42, 43]. Other observations suggest that the α1-ATS variant can also undergo loop-sheet polymerization and heteropolymerize with α1-ATZ, findings which provide a potential explanation for liver disease in PISZ patients [44, 45]. More recent studies by Huntington and colleagues elucidated the crystal structure of a stable serpin dimer and provided the basis for an interesting new model in which at least two different domain-swapping mechanisms explain the formation of polymers and insoluble aggregates of α1-ATZ [46–48]. This “domain swapping mechanism,” which has also been described for a number of other proteins, seems most consistent with the recent characterization of a putative ATZ monomer crystal structure [49], and also provides explanations for several previously recognized phenomena that have been somewhat mysterious: how the α1-ATZ molecule transitions from a relatively normally folded intermediate into polymers and insoluble aggregates; how a small proportion of newly synthesized α1-ATZ molecules (10–15%) are kinetically capable of folding into a conformation that can traverse the secretory pathway; and how accumulation of polymerized α1-ATZ in the ER does not activate the UPR, presumably because the structure of the polymer closely resembles the relaxed conformation of the normally folded monomer.
Two series of studies have suggested that polymerization of α1-ATZ is the cause of its retention in the ER, including, most notably, studies in which its secretion is partially corrected by introduction of a second mutation that suppresses loop-sheet polymerization [38]. However, those studies did not exclude the possibility that retention is caused by a distinct abnormality in folding that is partially corrected by the second, experimentally introduced mutation. Indeed, several recent observations militate against the idea that polymerization is the cause of ER retention. First, naturally occurring variants of α1-AT in which the C-terminal tail of α1-AT is truncated, including a double mutant with the substitution that characterizes the Z allele and the substitution that results in C-terminal truncation, are retained in the ER even though they do not polymerize [50]. Second, only approximately 18% of the intracellular pool of α1-ATZ at steady state is in the form of polymers in model cell lines characterized by marked ER retention [50, 51]. A greater degree of polymerization is thought to be prevented by the activity of ER chaperones. Most of the cellular pool of α1-ATZ in the ER in vivo is in heterogeneous soluble complexes with multiple ER chaperones (Figure 25.3) [51, 52], a state that cannot be modeled by studies of purified α1-ATZ in vitro. Taken together, these observations suggest the possibility that polymerization of α1-ATZ in the ER is an effect, rather than a cause, of the retention. This possibility does not preclude a specific role of polymers in the pathogenesis of tissue injury. In fact, three new lines of evidence argue for the specific role of polymers in injury to the liver. The first of these is the discovery that an inherited form of progressive dementia is associated with mutations in neuroserpin that have effects on the structure of that molecule that are almost identical to the effects of the Z mutation on α1-AT [53]. Second, there is now evidence suggesting that insoluble polymers and aggregates of α1-AT that accumulate in the ER at higher levels of expression are degraded by a distinct mechanism from that which occurs at lower levels of expression, i.e., autophagic as distinct from proteasomal degradation [54]. Third, recent studies indicate that accumulation of the polymerogenic mutant α1-ATZ activates cellular signaling pathways that are distinct from those activated by non-polymerogenic α1-AT mutants [54].
Figure 25.3 Diagram of distinct fates of wildtype α1-ATM vs. mutant α1-ATZ polypeptides. During/following (A) translation, α1-ATM is (B) bound by chaperones, which (C) facilitate normal protein folding, followed by (D, E) chaperone dissociation and vesicular transport out of the ER (and ultimately of the hepatocyte). Mutant α1-ATZ, which is (F) also chaperone bound, does not (G) fold or (H) access the secretory pathway normally. Instead, mutant α1-ATZ (I) aggregates and (J) monomers are targeted for degradation by the autophagolysosomal and ERAD pathways, respectively.
The distinction between misfolding vs. polymerization as the primary inciting event for intracellular accumulation and impaired secretion of α1-ATZ is important for consideration of potential therapeutic interventions. In either case, however, there is powerful evidence that the tendency for α1-ATZ to misfold, polymerize, and accumulate within the hepatocellular secretory pathway is fundamental to its proteotoxicity and liver-damaging effects.
Cellular Adaptive Responses to Mutant α1-Antitrypsin Z Retained in the Endoplasmic Reticulum
We now know that cells have elaborate mechanisms for degrading mutant proteins that are retained in the ER. The pathways by which retained α1-ATZ is degraded are also candidates for genetic variations that predispose some homozygotes or protect other homozygotes from liver injury by the gain-of-toxic function mechanism. One study has substantiated this concept by revealing a lag in ER degradation of α1-ATZ after gene transfer into cell lines derived from susceptible hosts, homozygotes with liver disease, when compared with cell lines from protected hosts, homozygotes without any liver disease [55].
Several pathways appear to be involved in the ER degradation of α1-ATZ. The proteasomal system was implicated first and has since been demonstrated in a number of model systems [54]. Both the classical ubiquitin-dependent and the ubiquitin-independent proteasomal pathways play a role. The mechanism by which molecules like mutant α1-ATZ that are found in the lumen of the ER reach the proteasome in the cytoplasm has been called ER-associated degradation (ERAD) and involves some type of retrograde translocation mechanism. A multiprotein complex that includes luminal and membrane-bound chaperones, membrane-spanning transport proteins, and cytoplasmic ubiquitin ligases has been implicated in the ERAD pathway for model luminal ERAD substrates. A mechanism in which the proteasome directly mediates extraction of substrates from the ER membrane is also favored as a part of the mechanism by which ERAD mediates its actions. Nevertheless, there was evidence from studies of the proteasomal pathway in mammalian cell lines, cell-free microsomal translocation systems, and yeast that this pathway could not completely account for disposal of α1-ATZ.
Autophagy was implicated as a second major pathway for ATZ disposal in α1-AT deficiency when a marked increase in autophagosomes was observed in fibroblast cell lines engineered for expression of α1-ATZ and increased autophagosomes were observed in the livers of ATZ-expressing PiZ mice and in liver biopsy specimens from PiZZ patients [23]. Autophagy is a catabolic process by which the cell digests internal molecules and organelles to generate amino acids as a survival mechanism. Autophagy also appears to play a role in homeostasis, cell growth, and differentiation. It is characterized by the formation of double-membrane structures, called autophagosomes, which subsequently fuse with lysosomes to degrade the enveloped contents. Therefore, the presence of abundant autophagosomes in hepatocytes from humans with, and model systems of, α1-AT deficiency raised the possibility that autophagy could be involved in degrading the mutant α1-ATZ that is retained in the ER. Definitive genetic evidence for the functional contribution of autophagy to α1-ATZ degradation was subsequently provided by studies showing delayed α1-ATZ disposal in α1-ATZ-expressing, autophagy-deficient (Atg5-null) mouse embryonic fibroblasts (MEFs [56]) and (Atg6- or Atg16-null) yeast strains [57]. Furthermore, in the ATG5-null MEF cells it became possible to observe massive accumulation of α1-ATZ with very large inclusions throughout the cytoplasm, while the yeast studies showed that delayed α1-ATZ degradation was most apparent in the autophagy-deficient strains when α1-ATZ was produced at high levels. Therefore, in addition to providing definitive evidence that autophagy contributes to the disposal of α1-ATZ, these studies also suggest that it plays a “homeostatic” role in the α1-AT-deficient state by preventing toxic cytoplasmic accumulation of α1-ATZ through continuous piecemeal digestion of insoluble aggregates. These studies also indicate that at lower levels of production α1-ATZ in the ER is predominantly soluble and can be degraded by the proteasome whereas at higher levels it is more likely to transition to insoluble polymers/aggregates that require autophagy for disposal. Studies in the yeast models also showed that a mutant subunit of fibrinogen that forms insoluble aggregates in the ER of liver cells in an inherited form of fibrinogen deficiency depends on autophagy for disposal [58]. This type of fibrinogen deficiency has been associated with a chronic liver disease characterized by distinct fibrillar aggregates in the ER of liver cells. Thus, these results substantiate the concept that chronic liver disease can be caused by accumulation of an aggregation-prone protein in the ER and that autophagy is specialized for the disposal of aggregation-prone proteins that accumulate in the ER [58].
Several lines of evidence suggest that there are one or more other pathways that contribute to disposal of α1-ATZ. For example, tyrosine phosphatase-dependent- [59] and sortilin-mediated- [60] pathways have been reported. The studies of Kruse et al. [57] indicated that α1-ATZ could be transported to the trans-Golgi and then targeted to the lysosome of yeast cells. Another pathway for ATZ disposal that diverges from canonical autophagy was recently identified in a powerful C. elegans model of α1-AT deficiency and found to be present in a mammalian cell line model as well [61]. This pathway is particularly interesting because it is suppressed by insulin signaling and upregulated by disrupting insulin signaling, and when upregulated that way completely mitigates ATZ proteotoxicity.
In addition to degradation mechanisms, cells appear to have a number of other mechanisms by which they attempt to adapt to, or protect themselves from, proteins that are retained in the ER. Because differences in an adaptive response could theoretically explain variation in the liver disease phenotype, we have recently begun a series of studies designed to characterize how cells respond to ER retention of α1-ATZ using cell line and transgenic mouse model systems with tetracycline-inducible expression of the mutant gene. These models permit us to see the earliest responses and to separate them from compensatory adaptations that arise later. Because the relative expression of the mutant gene can be regulated in this kind of model system, it is also possible to determine the effect of the mutant gene product at specific concentrations, at specific stages of development, and for specific intervals.
Accumulation of α1-ATZ in the ER is sufficient to activate the autophagic response. This was demonstrated by mating two types of transgenic mice: the Z mouse, with liver-specific inducible expression of α1-ATZ in which α1-ATZ accumulates in the ER of hepatocytes only when doxycycline is removed from the drinking water [56], and the GFP-LC3 mouse [62], in which autophagosomes have green fluorescence because LC3 is an autophagosomal membrane-specific protein. Although green fluorescent autophagosomes are only seen in the liver of the GFP-LC3 mouse when it is starved, they are seen in the liver of the Z × GFP-LC3 mouse simply by removing doxycycline from the drinking water and inducing the accumulation of mutant α1-ATZ in the ER of hepatocytes [56]. This is a particularly important observation because it represents the first evidence that a single protein can activate the autophagic response. Although there is much still to learn about how accumulation of α1-ATZ in the ER activates autophagy, recent studies have shown that the regulator of G protein signaling 16 (RGS16) may play a role in mediating this effect. This regulator is an antagonist of Gαi3, which ordinarily inhibits hepatic autophagy [54]. Hidvegi et al. [63] showed that RGS16 is markedly upregulated when α1-ATZ expression is induced and that this effect could de-repress the autophagic response.
Surprisingly, accumulation of α1-ATZ in the ER has minimal activating effects on the UPR. The UPR is a signaling pathway that activates a number of genes in response to accumulation of unfolded proteins in the ER [54]. In addition to new synthesis of ER chaperones, such as BiP (GRP78), and enzymes that facilitate disulfide bond formation, other processes occur, including bolstering the protein-folding capacity of the ER, increasing lipids for synthesis of new ER membrane required to handle the increased protein load, and increasing synthesis of proteins that participate in degradative and other cellular translocation mechanisms. There is also a decrease in initiation of translation in such a way that only specific mRNAs can be translated, thereby preventing the de novo synthesis of proteins that will further accumulate in the ER [54]. Although we do not detect activation of the UPR when α1-ATZ accumulates in the ER, we do detect it when truncated non-polymerogenic α1-AT variants accumulate in the ER [64], suggesting that the failure to activate the UPR is specific for the α1-ATZ variant [64]. With what is known about the mechanisms by which the UPR is initiated, it has always been relatively easy to understand how polymerized or aggregated proteins would not elicit this response. Results of structural studies provide an explanation for why soluble monomeric α1-ATZ might not be recognized by the UPR sensing apparatus [38]. These results suggested that the monomeric α1-ATZ intermediate adopts a conformation that resembles the wild-type molecule and, therefore, would not be recognized as unfolded. The lack of UPR signaling in cells that accumulate α1-ATZ is likely to be an important part of how liver cells behave in vivo in α1-AT-deficient-patients, perhaps partially accounting for the chronic, slowly progressing nature of the disease. A liver picture dominated by hepatocyte apoptosis and fulminant liver failure would be likely if the UPR was being activated.
In contrast, the ER overload pathway and its signature target nuclear transcription factor kappa B (NF-κB) is activated when α1-ATZ accumulates in the ER [64]. The ER overload pathway was first described as activation of NF-κB in response to the type of “ER stress” that is generated by treatment of cells with brefeldin A or by experimental accumulation of adenovirus E3 protein in the ER [64]. The fact that ER accumulation of α1-ATZ activates NF-κB but not the UPR provides strong corroborating evidence for the previously held contention that the ER overload pathway was distinct from the UPR. Activation of NF–κB has potentially important implications for target organ injury in α1-AT deficiency. In particular it plays an important role in inflammation-associated carcinogenesis and this appears to be related to its effect on cell proliferation [54]. In recent studies in which the PiZ mouse was mated to mouse models engineered for absence of NFκB signaling we found more severe inflammation, fibrosis, steatosis, dysplasia, and more hepatocytes with globules [65] indicating that NFκB signaling protects the liver from the effects of α1-ATZ accumulation. Interestingly the effect of NFκB signaling under these circumstances is through a small number of downstream target genes: Egr-1, a transcription factor that is essential for liver regeneration [66]; RGS16, the inhibitor of G-protein signaling implicated in activation of autophagy [63] and mentioned above; and matrix metalloproteases MMP7 and MMP12. Each of these targets appear to mediate parts of the hepatic response to α1-ATZ accumulation: decreased proliferation of hepatocytes with massive ATZ accumulation, known as globule-containing hepatocytes, due to Egr1-downregulation; increased autophagy due to RGS16 upregulation; and counteracting of fibrogenesis by MMP7 and MMP12 upregulation.
Using cell line and mouse model systems with inducible expression of mutant α1-ATZ two other signal transduction pathways associated with ER stress have been investigated. Accumulation of α1-ATZ in the ER led to cleavage and activation of the ER caspases: caspase-12 in mouse cells and caspase-4 in human cells [64]. These results indicate that both the mitochondrial and ER caspase pathways are activated in α1-AT deficiency. Accumulation of α1-ATZ in the ER also specifically mediated cleavage and activation of BAP31 (B-cell receptor-association protein 31) [64], an integral membrane protein of the ER that is involved in the ER retention of several proteins and appears to mediate proapoptotic signals from the ER to mitochondria. This last point may provide a mechanistic basis for the mitochondrial dysfunction that has been found in cell line and transgenic mouse models as well as in the liver of α1-AT-deficient patients [67].
Genomic analysis of the liver from our mouse model with inducible expression of mutant α1-ATZ demonstrated several other cellular response pathways that are specifically activated, including the lipogenic and sterolgenic signaling pathways that are mediated by transforming growth factor-β (TGFβ), extracellular regulated kinase 2, and the sterol regulatory element-binding proteins [63]. The TGF-β signaling pathway plays a dominant role in tissue fibrosis and can explain the primary pathological effect of α1-ATZ accumulation on the liver. Related to this consideration, we also recently found that accumulation of the α1-ATZ variant in respiratory epithelial cells elicits fibrosis in the lungs, with evidence for fibrosis in the lungs of α1-AT deficiency patients with very severe COPD [68]. The mechanism by which accumulation of misfolded proteins in the ER elicits TGFβ signaling has not been studied.
c-Jun N-terminal kinase activation was recently demonstrated in the liver of the PiZ mouse model, as well as in liver specimens from patients with α1-AT deficiency [69]. Interestingly this signaling effect leads to increased expression of α1-ATZ and therefore likely plays a role in amplifying the pathobiology of misfolded protein accumulation.
Modifiers that affect the function of any of these pathways could increase susceptibility to liver disease according to our theory. Indeed, a single nucleotide polymorphism (SNP) in the downstream flanking region of the Man1b1 ER mannosidase I gene has been implicated in early-onset liver disease among α1-AT-deficient individuals [70]. Because ER mannosidase I plays a role in ERAD, a polymorphism that affects its function would be a prime candidate for a modifier of liver disease in α1-AT deficiency. In subsequent studies, the identified variant in Man1b1 was shown to reduce intracellular levels of the mannosidase [71], and recent experiments have also shown that the MAN1B1 protein is actually localized to the Golgi and plays a role in regulation of protein secretion as a part of the protein quality control network recently recognized to be localized in the Golgi [72]. Those experiments also provided a basis for how reduced levels of MAN1B1 could theoretically lead to greater intracellular α1-ATZ accumulation [72]. Taken together, these studies appear to validate our hypothesis that intracellular degradation pathways are targets of liver disease modifiers, but further population studies of this variant would be reassuring [73, 74].
A SNP in the upstream flanking region of the gene for α1-AT itself has also been implicated in liver disease susceptibility [75]. However, the nature of that variant could not be reconciled with how it might affect liver disease susceptibility and its statistical association with variation in the liver disease phenotype was dependent on a questionable classification of population sub-groups.
Our hypothesis for variation in liver disease susceptibility also identifies signaling pathways that could increase or decrease α1-ATZ proteotoxicity as potential targets for disease modifiers. Although we have not encountered an example of this potential scenario, we expect future studies will identify such a mechanism.
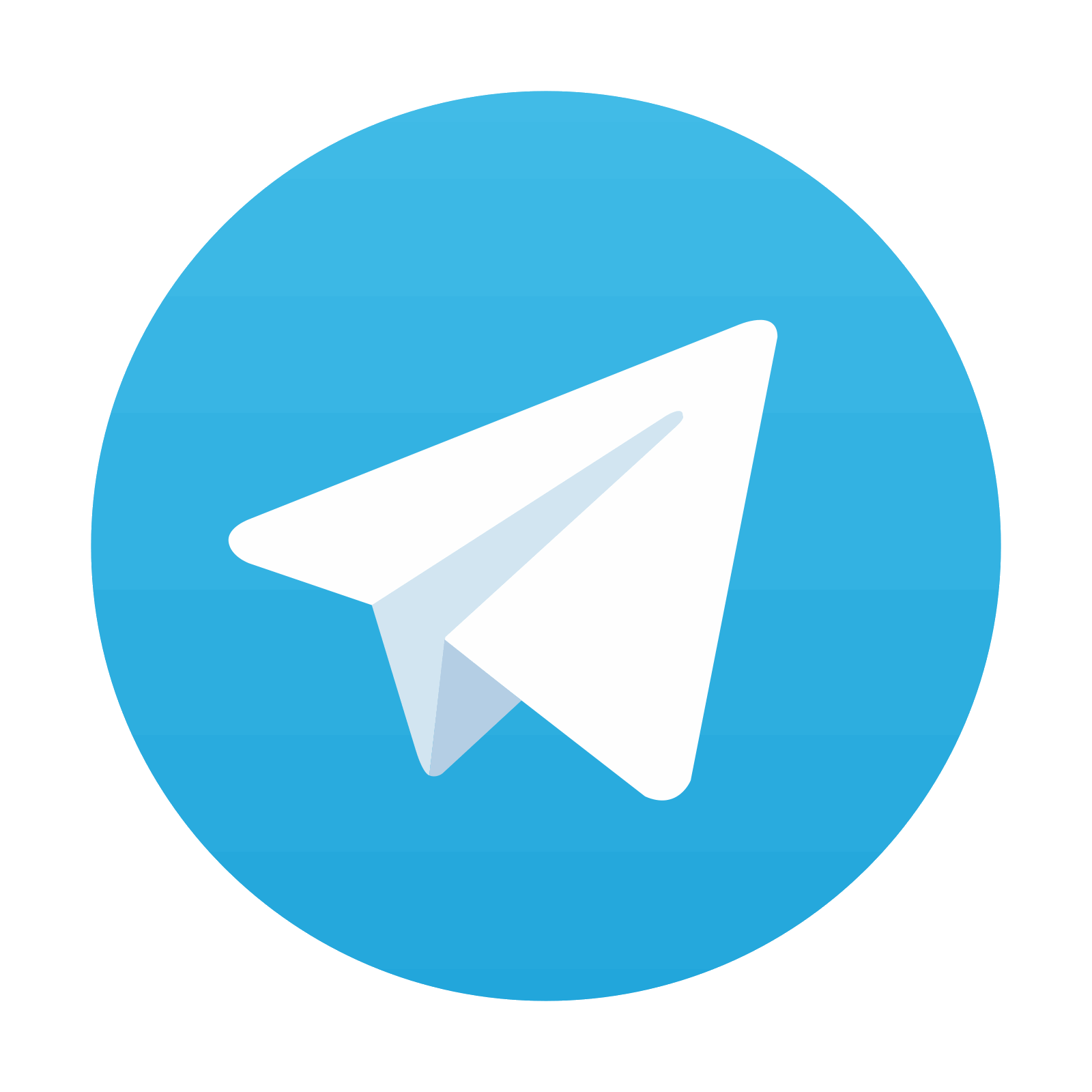
Stay updated, free articles. Join our Telegram channel
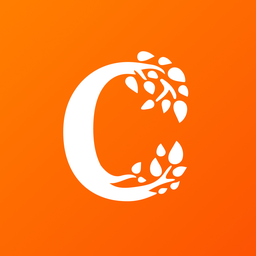
Full access? Get Clinical Tree
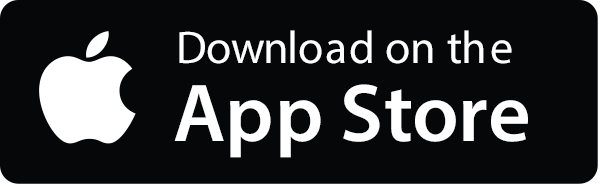
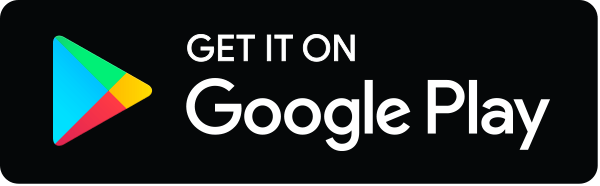
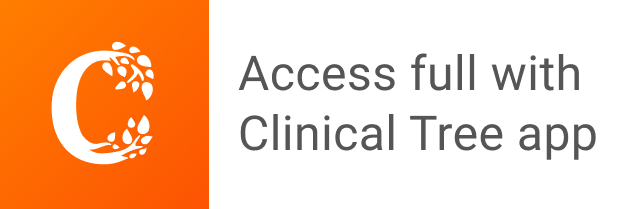