Abstract
Liver disease due to inborn errors of metabolism (IEM) is relatively common, and this group of disorders is recognized with increasing frequency as a cause of disease at every life interval from the fetus to the geriatric patient. The specific patterns of disease, however, overlap with many other causes of liver disease. Specific testing for metabolites, or more recently through genomic sequencing, is required to appropriately diagnose these metabolic conditions, which increasingly have specific and effective treatments. The collective incidence of IEMs is often underestimated, and diagnostic errors often occur, leading to devastating consequences for patients and their families, including several historical examples of criminal charges against parents for child abuse and neglect [1].
Introduction
Liver disease due to inborn errors of metabolism (IEM) is relatively common, and this group of disorders is recognized with increasing frequency as a cause of disease at every life interval from the fetus to the geriatric patient. The specific patterns of disease, however, overlap with many other causes of liver disease. Specific testing for metabolites, or more recently through genomic sequencing, is required to appropriately diagnose these metabolic conditions, which increasingly have specific and effective treatments. The collective incidence of IEMs is often underestimated, and diagnostic errors often occur, leading to devastating consequences for patients and their families, including several historical examples of criminal charges against parents for child abuse and neglect [1].
Many IEMs are the result of deficiency of enzymes that are primarily expressed in the liver, but may lead to symptoms through systemic effects caused by accumulation, or deficiency, of the product of the enzyme. These are primarily inborn errors of the intermediate metabolism of amino acids, carbohydrates, and fatty acids, and include the urea cycle defects. In most cases, hepatocellular injury is rare, although it is more common with several specific disorders that appear to accumulate chemicals that are toxic to hepatocytes, including tyrosinemia type I and some urea cycle disorders, which may lead to liver failure or hepatocellular carcinoma. Primary defects of bile acid synthesis, cholesterol synthesis, metal transport, peroxisomal biosynthesis or congenital disorders of glycosylation may cause hepatocellular injury. Other IEMs may lead to the storage of fat or complex molecules in the hepatocytes causing massive hepatomegaly with relatively little injury to the hepatocytes themselves, including many of lysosomal storage diseases and mucolipidoses. Finally, defects in mitochondrial energy metabolism may have complex effects on the liver. Clinical aspects of most of these conditions are discussed elsewhere in this book; the focus of this chapter is on the biochemical laboratory diagnosis of the major groups of metabolic diseases affecting the liver.
The inborn errors listed in Table 24.1 share a common natural history, which is the occurrence of either acute life-threatening illness in early infancy or unexplained developmental delay with intercurrent episodes of metabolic decompensation in later childhood. Unfortunately, the clinical presentations of these diseases are often attributed to a variety of other causes, including:
accidental ingestion
cerebral palsy
child abuse (including factitious disorder by proxy syndrome)
cyclic vomiting syndrome
developmental delay
intraventricular brain hemorrhage
Reye syndrome
seizure disorder
Sepsis
sudden infant death syndrome
sudden unexpected death in early life (attributed to infections).
Table 24.1 Most common inborn errors of metabolism associated with acute life-threatening illness
Type of Disorder | Types/Examples |
---|---|
Organic acidurias | Defects of branched-chain amino acid metabolism |
Congenital lactic acidemias | Pyruvate oxidation defects Gluconeogenesis defectsKrebs cycle defectsRespiratory chain defects |
Disorders of fatty acid transport and oxidation | Defects of membrane-bound enzymes and transportersDefects of mitochondrial matrix enzymes |
Urea cycle disorders | Ornithine transcarbamylase, carbamylphosphate synthetase deficiency |
Amino acid disorders | Maple syrup urine disease Non-ketotic hyperglycinemia |
In the past, after a patient was properly diagnosed with a metabolic condition, it was not uncommon to find that an older sibling had presented with similar symptoms but had passed away without a precise diagnosis. Tragically, these cases occasionally had been misdiagnosed as poisoning or intentional injury, and some parents had had to endure criminal prosecution. The wide-spread availability of sensitive testing for unusual metabolites discussed below has made this type of diagnostic misadventure exceedingly rare now. Also, the expansion of newborn screening in the mid to late 1990s greatly enhanced the early diagnosis of many of these conditions.
It is critical for the healthcare provider to remember that newborn screening does not identify all IEMs that affect the liver, nor is it 100% sensitive for every disorder. Because symptomatic, diagnosis is more rare due to many disorders being identified by newborn screening, providers must be even more attentive to the possibility of an IEM to prevent further, unnecessary, delay in diagnosis.
Two major misconceptions stand behind the frequent failure of correctly diagnosing a metabolic disorder: (1) that a high degree of specialization is needed in order to suspect and initially manage acutely ill patients with inborn errors of metabolism, and (2) that the conditions are rare, therefore they are unlikely. Because the conditions are rare, specific training in metabolic genetic diseases receives marginal coverage in the curriculum of medical students and pediatric residents at many academic institutions. This situation is disconcerting because newborns and infants with life-threatening illnesses of the type covered here are initially placed under the responsibility of either a general practitioner or a pediatric subspecialist, and rarely immediately into the hands of a metabolic specialist. Each individual IEM is indeed rare, however, taken together, they are relatively less rare, and in the setting of unexplained, acute life-threatening illness in childhood, they are as common as many conditions that are routinely considered in the diagnosis.
The aim of this chapter is to emphasize that a methodical use of routine laboratory tests supplemented by targeted specialized investigations should allow a prompt biochemical diagnosis of patients affected with one of these metabolic diseases, preventing serious sequelae or almost inevitable death.
A growing number of these diseases can be treated effectively and efficiently, and new modalities of therapy promise to expand that number. Therefore, high morbidity and mortality should not be systematically assumed in reference to these inborn errors of intermediate metabolism.
Recognition of Signs and Symptoms
The clinical aspects of metabolic disorders with primary hepatic disease are specifically addressed elsewhere in this book. The diagnostic process for IEM begins with a high level of suspicion, thus a brief recapitulation of the cardinal clinical findings of these disorders is necessary to put the laboratory analyses to be described in a practical perspective.
Although the final diagnosis of a specific inborn error of metabolism is a laboratory process, several clinical elements should effectively raise the level of suspicion in that diagnostic direction. Saudubray [2] delineated several pathophysiologic patterns of clinical presentation, the recognition of which may provide valuable diagnostic signs. Even though infants have a relatively limited palette of symptoms to demonstrate a wide variety of severe illnesses (e.g., IEM, sepsis, encephalitis, ingestion, Reye syndrome, and others) the clinical picture in a newborn or infant that is dominated by severe neurologic deterioration is more informative than might be assumed. In the neonatal period, a patient who becomes rapidly comatose after a variable (hours to days) symptom-free period could be clinically categorized as being in an intoxication-type of neurologic distress. By comparison, the absence of a symptom-free period associated with a delayed evolution of coma is rather indicative of an energy-deficit type of neurologic distress. In both cases, a failure to respond to symptomatic therapy, followed by rapid deterioration of an infant’s general status, is a typical manifestation of metabolic disease. Many organic acidemias, urea cycle disorders, and selected disorders of amino acid metabolism belong to the former category, and primary lactic acidemias, and severe forms of mitochondrial fatty acid oxidation (FAO) disorders pertain to the latter. Additional stratification of clinical symptoms can also be beneficial: the presence of cardiac failure and other functional heart disorders are characteristic for FAO disorders and mitochondrial diseases. Persistent hypoglycemia and liver dysfunction as the most prominent findings suggests a possible disorder of carbohydrate metabolism, such as glycogenosis type I or III, fructose intolerance, or galactosemia. These disorders are discussed elsewhere in this book.
Routine Laboratory Investigations
Table 24.2 lists the basic laboratory investigations that are recommended when a clinical picture compatible with an inborn error of metabolism has been recognized. Recognition of a diagnostic pattern, potentially within hours of the time of admission, can direct implementation of adequate therapeutic measures pending confirmation of the preliminary diagnosis by specialized laboratory investigations [3]. Although blood gases, electrolytes, and glucose are routinely part of the evaluation of any acutely ill child, ammonia, lactate, and pyruvate are not consistently requested at admission, but they should be. In urine, the qualitative determination of 3-keto acids by a commercial dip strip (e.g., Chemstrip; Roche Diagnostics, Basel, Switzerland) and 2-keto acids by the dinitrophenylhydrazine (DNPH) test can be performed rapidly in the early stage of evaluation of an acutely ill patient, although the reagents for the DNPH test are no longer kept in most hospital laboratories. A guideline for diagnostic pattern by routine laboratory investigations is shown in Table 24.3 and discussed in the context of each specific group of disorders. Obviously, partial deviations from a model pattern are always possible, in light of the variable nature of a particular metabolic defect, and the role that environmental factors play in individual patients.
Table 24.2 Routine laboratory investigations in the initial evaluation of patients with inborn errors of metabolism
Sample | Assays |
---|---|
Serum |
|
Urine |
|
Table 24.3 Interpretation of routine laboratory investigations for the diagnosis of inborn errors of metabolism
Organic acid disorders | Primary lactic acidemias | Amino acid disorders | |||||||
---|---|---|---|---|---|---|---|---|---|
Organic acidemias | Fatty acid oxidation disorders | Pyruvate oxidation | Gluconeogenesis | Pyruvate carboxylase | Respiratory chain | Urea cycle disorders | MSUD | NKH | |
Approximate number of IEMs | >50 | 23 | 7 | 3 | 3 | >100 | 8 | 4 | 1 |
Neurologic distress | I | ED | ED | ED | ED | ED | I | I | I |
Metabolic acidosis | +++ | + | +++ | +++ | +++ | +++ | – | – | – |
3-Keto aciduria (ketone bodies) | +++ | – | – | + | ++ | ++ | – | + | – |
2-Keto aciduria (DNPH positive) | – | – | +++ | – | + | – | – | +++ | — |
Hyperammonemia | + | + | + | + | +++ | + | +++ | – | =- |
Hypoglycemia | + | +++ | – | +++ | + | + | – | – | = |
Lactic acidemia | + | + | +++ |
| +++ | +++ | – | – | |
Lactate:pyruvate ratio | <15 | >20 | >30 | >50 | |||||
3- Hydroxybutyrate/ acetoacetate ratio | >2 | >2 | <1 | >3 |
DNPH, dinitrophenylhydrazine; IEM, inborn error of metabolism; MSUD, maple syrup urine disease; NKH, non-ketotic hyperglycinemia; I, intoxication type; ED, energy deficiency type of neurologic distress; +, possibly present; +++, typically present with high diagnostic significance; –, not typically present.
Other simple manual tests that could aid the differential diagnosis of other disorders in acutely ill patients include the detection of reducing substances and sulfites in urine. A positive reducing substances test (formerly sold as Clinitest, Bayer AG, Leverkusen, Germany) may be indicative for galactosemia or hereditary fructose intolerance, however, most laboratories have discontinued the test because the reagents have not been available for several years. Gross galactosuria, however, also may occur in patients with severe liver disease of any origin, and false negative reactions for reducing substances have been observed in symptomatic newborns with galactosemia [4]. Qualitative detection of sulfites in fresh urine from a patient presenting with severe neurologic distress, lactic acidosis, and hypouricemia is indicative of a diagnosis of molybdenum cofactor deficiency, which is also typically, but not always, associated with serum concentrations of homocysteine and uric acid that are below the reference interval for age.
Specialized Laboratory Investigations
Table 24.4 lists the methods most often used for measuring metabolites pertinent to the diagnosis and management of IEMs affecting the liver. It should be recognized that the abnormal metabolic patterns in the acute phase (especially those seen in organic acid disorders) are often pronounced and may be different from the pattern during the chronic phase. These analytes and methods may differ at different laboratories.
Table 24.4 Common methods of analysis for inborn errors of metabolism
Method | Assays | Principle | Comment |
---|---|---|---|
Gas chromatography – mass spectrometry (GC-MS) | Urine organic acids, urine orotic acid, urine succinylacetone, etc. | Separated on polar stationary phase and identified by a mass fragmentation detector | Most specific and sensitive; used in referral centers |
Tandem mass spectrometry (MS/MS) | Acylcarnitine profile and free and total carnitine (& newborn screening) | Compounds are separated by molecular weight then fragmented and detected based on the specific daughter compounds resulting from high energy fragmentation | High molecular specificity and detection sensitivity |
Liquid chromatography – ion exchange chromatography (LC-IEC) | Plasma amino acids (AAs), urine AAs, CSF AAs, etc. | Compounds are separated by liquid chromatography and detected by retention time (flow rate) via light absorption | Excellent for quantitation with high molecular specificity and detection sensitivity |
Liquid chromatography – tandem mass spectrometry (LC-MS/MS) | Total and free carnitine, acylcarnitine profile | Compounds are separated by high performance liquid chromatography and detected by molecular weight analysis using MS/MS | Excellent for quantitation |
Tissue enzymatic assays | Respiratory chain, lysosomal enzymes, etc. | Kinetic assays | Requires skilled technician; sensitivity varies with quality of sample and preparation of sample |
Table courtesy of Hoanh Nguyen, MD.
On admission, special consideration should be given to the immediate collection and proper storage of urine and blood samples from patients in severe decompensation. These samples may not be available post-mortem, or material collected even after a partial recovery may not show certain diagnostic abnormalities otherwise detectable, or more easily detectable, under acute conditions. Any volume of urine (stored at –20°C with no preservatives, or even a wet diaper obtained at the emergency room) and plasma/serum (stored at –20°C) should be considered adequate for testing. Alternatively, a blood spot on filter paper could provide enough material for one or more of the specialized laboratory investigations described later in this chapter. In case of death, collection of body fluids, including bile, and tissues should be secured as soon as possible.
Quantitative amino acids, free and total carnitine, and quantitative or qualitative acylcarnitines in plasma, as well as urine organic acids and acylglycine analysis, together will lead to a biochemical diagnosis for the vast majority of the acute, intoxication type disorders. There are, of course, indications, advantages, and limitations to these tests in the differential diagnosis of each group of inborn errors of metabolism. Furthermore, the provision of a detailed interpretation is essential because a biochemical genetics service differs from a conventional clinical chemistry laboratory in the expectation to provide an overview of abnormal and relevant negative results (i.e., ketotic vs. non-ketotic dicarboxylic aciduria, or methylmalonic aciduria with or without homocystinuria), to quantify pertinent abnormal compounds whenever possible, correlate available clinical information, explain the elements of the differential diagnosis, provide recommendations for additional biochemical testing and in vitro confirmatory studies (enzyme assay, molecular analysis), give the name and phone number of key contacts who may provide these studies, and provide a phone number for the laboratory director in case a referring physician has additional questions.
DNA Sequencing Technologies
Advances in DNA sequencing technologies are having a profound impact on the laboratory diagnosis of IEMs and other genetic disorders causing liver disease. Next generation sequencing (NGS) techniques refer to a variety of chemistries used for DNA sequencing. Until recently these approaches used the concept of high-throughput massively parallel sequencing to sequence millions of short copies, or “reads,” of DNA, often after selection of the fragments to be sequenced by some targeted capture method. The millions of short “reads” of sequence would be aligned by computer algorithms, allowing the longer genetic sequence to be pieced together. This powerful approach sequenced the same regions dozens, or even hundreds, of times, allowing for confidence in the accuracy of the result. Newer methods allow for much longer reads, and will again bring new advantages, and challenges, to the field.
Exome sequencing refers to the simultaneous sequencing of the entire coding region, or exons, of every gene. This accounts for 2–3% of the entire genome. Genomic sequencing refers to sequencing of the entire genome. Sequencing of the exome requires some sort of capture method, which can introduce some bias into the process, and can lead to certain exons being systematically missed. Genomic sequencing typically does not have a capture or selection step, and thus has better coverage. Unfortunately, there remain major limitations on the ability to interpret the significance and meaning of all sequence variants in both exomes and genomes, thus, diagnostic findings may be missed, and there may be variants of unknown significance that cannot be accurately determined to be benign or disease causing. Large deletions or rearrangements of DNA also may be missed by these techniques, although algorithms to determine these structural, or copy number variants, from sequencing data are improving rapidly.
DNA sequencing is a powerful tool, but one major limitation is that when DNA testing does not find a causative variant, it is impossible to know whether there is not a variant to be found, or whether the technology was inadequate to find the causative variant. Thus, a “negative” DNA result can rarely completely rule out a diagnosis, although it can markedly reduce the likelihood of a specific diagnosis. This is especially true for recessive disorders, where even finding one copy of the gene with a deleterious variant would markedly increase suspicion.
These technologies continue to advance rapidly, and it is likely that they will continue to become more and more the first-line approach to diagnosis of IEMs affecting the liver [5].
Laboratory Evaluation of Inborn Errors of Metabolism that Present with Acute or Chronic Liver Disease
Inborn errors of metabolism are among the most common causes of acute hepatic failure in children [6], with evidence of hepatocellular injury and synthetic function defects. Basic metabolic screening tests are appropriate to begin with, and may point to some of the conditions only rarely present with hepatic failure discussed below. These can include urea cycle disorders, organic acidemias and fatty acid oxidation defects.
Defects in carbohydrate metabolism, particularly galactosemia and hereditary fructose intolerance (HFI), can present with primary hepatic failure. Galactosemia is included in most state’s newborn screening panels, however, as with any screening test, cases have been missed, therefore clinicians need to maintain a high index of suspicion. Diagnosis of galactosemia due to defects in galactose-1-phosphate uridyl transferase is confirmed by measuring the enzyme activity in red blood cells. Whole red cell lysates are used to measure the characteristic metabolite, galactose-1-phosphate. Total galactose is also elevated, and is a marker for other defects in the pathway that do not typically cause liver dysfunction.
Hereditary fructose intolerance can cause severe, acute liver failure when fructose is introduced into the diet. Classically, this condition has been considered to not present until weaning when fruits are introduced, however, the addition of sucrose to a variety of sources of infant nutrition, particularly infant formulas marketed to be “easy” on the stomach, means that it should now be considered at any age. Hypoglycemia after feeding with sucrose is typical, but high index of suspicion should lead to DNA sequencing of the aldolase B gene (ALDOB) [7].
Defects in mitochondrial DNA replication, the so-called mitochondrial depletion syndromes, especially defects in DNA polymerase γ (POLG), may present with hepatic failure in infancy as part of the hepatocerebral phenotype. POLG causes Alpers syndrome, typically also presenting with neurological abnormalities, but others, including deoxyguanosine kinase (DGUOK) deficiency, MPV17, and many others, may have more or less neurological involvement early on. Lactic acidosis, with an increased ration of lactate to pyruvate (>20) indicating inadequate oxidation of NADH, may be seen in respiratory chain disease, but is neither completely sensitive nor specific. Elevated plasma alanine, formed from the transamination of excess pyruvate by the ALT enzyme, may indicate persistently elevated lactate and pyruvate. Liver biopsy for quantitation of mitochondrial DNA is diagnostic later in the course of these disorders but may not be adequately sensitive early on, therefore early DNA sequencing of a panel of genes causing mitochondrial DNA depletion is the most appropriate method to reach a diagnosis at this time.
Tyrosinemia type I causes hepatorenal failure due to deficiency of an enzyme fumarylacetoacetate hydrolase (FAH), several steps downstream from tyrosine in its degradation pathway. Tyrosine eventually accumulates, but the diagnostic marker succinylacetone is increased from birth. Assay of succinylacetone typically is performed by LC-MS and is part of the newborn screening in most states. Because the disease is easily treatable with a drug, nitisinone, that blocks an earlier step in the pathway [8], it is crucial to repeat testing even if newborn screening was normal.
Neonatal hemochromatosis, now recognized to be most commonly, but not exclusively, due to gestational autoimmune liver disease (GALD) [9] is discussed in Chapter 29, but recognized by the characteristic large increase in ferritin concentrations. This disorder is unrelated to hereditary hemochromatosis, a genetic predisposition to excessive iron absorption that is a not an uncommon cause of hepatic failure in adults.
Later in childhood, classical conditions such as α-1 antitrypsin deficiency or Wilson disease may present with a more progressive, chronic liver insufficiency pattern. Measurement of α-1 antitrypsin isoforms and DNA testing are diagnostic for the former. Wilson disease, due to autosomal recessive deficiency of ATP7B, is screened by measurement of serum copper (low in affected individuals) and ceruloplasmin (typically <20 mg/dL in affected individuals). Ceruloplasmin concentrations vary by age, so confirmation that the testing lab has appropriate age-specific cut-off ranges is necessary. Urinary copper excretion is typically increased in Wilson disease. Serum copper and ceruloplasmin do not have 100% sensitivity, so are good for screening in the setting of a low likelihood of disease, but if the pre-test probability of Wilson is high, for instance in an older teen with Kayser-Fleischer rings of the cornea, DNA testing is useful.
This list is not complete, with other rare causes including Niemann-Pick disease, some congenital disorders of glycosylation (CDG), and others having been reported as the cause of hepatic failure. The CDGs in particular can be difficult to diagnose, and are often not recognized until broad-based genomic testing is requested.
Primarily Cholestasis
Some of the IEMs that present with more chronic hepatocellular dysfunction were discussed above, namely Wilson disease and α-1 antitrypsin deficiency. Other considerations of conditions that present primarily with cholestasis include defects of bile acid synthesis, progressive familial intrahepatic cholestasis, Rotor and Dubin-Johnson syndromes. Bile acid synthesis is part of the sterol synthetic pathway and has many possible defects. Screening tests of bile acids in plasma using a variety of chromatographic-MS methods are available, and sterol profiling using similar methods can help to pinpoint the diagnosis when inadequate bile acids are found on screening. Like many conditions discussed here, however, DNA sequencing approaches are gaining popularity because of their efficacy, relatively reasonable speed in making a definitive diagnosis, and accessibility. Many of these conditions, including cerebrotendinous xanthomatosis, are treatable with bile acid precursor supplementation, so a high index of suspicion and low threshold for testing is required [10].
Byler disease, due to a variety of ATP-dependent phospholipid transporters, typically presents with persistent cholestasis, and later progressive cirrhosis and liver failure. Diagnosis is typically made by DNA testing of a panel of genes [11] or by genomic sequencing. Rotor and Dubin-Johnson syndromes both present with conjugated hyperbilirubinemia secondary to defects in several trans-membrane transporters for anionic compounds. Diagnosis can be facilitated by quantitation of urinary excretion of coproporphyrin I, or by liver biopsy, but DNA testing appears to be now a more expeditious approach.
Primarily Hepatomegaly with Intact Hepatocellular Function
A number of IEMs cause significant hepatomegaly without significant hepatocellular injury or dysfunction. The lysosomal storage diseases, glycogen storage diseases, some congenital disorders of glycosylation, and some cholesterol trafficking defects present this way. The latter category includes cholesterol ester storage disease, the more severe form of which is referred to as Wolman disease. These conditions are due to defects in liposomal acid lipase (LIPA), and diagnosis is suspected when vacuolated lymphocytes are seen in peripheral blood, and foam cells are found on bone marrow biopsy. DNA testing confirms the diagnosis.
A full discussion of the diagnosis of lysosomal storage diseases is beyond the scope of this chapter. Typically, these defects are due to deficiencies in enzymes needed for the degradation of complex molecules, often components of membranes, and include lipids, complex carbohydrates or peptides. The presence of splenomegaly along with hepatomegaly is particularly suspicious. Many have other extra-hepatic manifestations that may suggest a specific diagnosis. The final diagnosis is typically made by panels of enzyme analyses from white blood cells or fibroblasts, but, more recently is shifting toward DNA sequencing panels as an earlier, if not first, diagnostic maneuver [12].
The glycogen storage diseases (GSD) are a group of disorders caused by deficiencies of one of several enzymes required for the release of glycogen stores from liver and muscle. The prototypical defect is glycogen storage disorder Ia caused by deficiency of glucose-6-phosphatase, the enzyme necessary for the final step of the release of glucose from glycogenolysis and gluconeogenesis. Hypoglycemia is persistent, with lactic acidosis, hyperuricemia and hypertriglyceridemia resulting from various mechanisms of dealing with excess metabolites that accumulate in liver due to the block. The GSDs that present with hepatomegaly and prominent hypoglycemia include types Ia, Ib, III, and sometimes VI, IX and XI (also called Fanconi-Bickel syndrome). GSD types IV and VIII, and sometimes VI, IX, XI may present with hepatomegaly without prominent hypoglycemia. The so-called GSD type 0, glycogen synthase deficiency, as one might expect does not cause hepatomegaly, but does present with hypoglycemia [13]. Definitive diagnosis of all of the GSDs presenting with hepatomegaly is made by enzyme assay from liver biopsy or DNA sequencing.
Laboratory Evaluation of Defects of Hepatic Enzymes that Present with Acute Illness, Metabolic Decompensation or Neurological Crisis
Organic Acidurias
Organic acids are water-soluble compounds containing one or more carboxyl groups as well as other functional groups (e.g., keto, hydroxy) and form the intermediate metabolites of all major groups of organic cellular components: amino acids, lipids, carbohydrates, nucleic acids, and steroids. Organic acidurias are a biochemically heterogeneous group of inborn errors of metabolism biochemically characterized by the accumulation of metabolites that either are not present in the circulation under physiologic conditions or are pathologic amounts of normal metabolites. Occasionally, a diagnostic compound formed from activation of alternative pathways in response to the loss of function of a specific gene product may be identified, for example succinylacetone in tyrosinemia type I, or alloisoleucine in maple syrup urine disease. The organic acidemias share a common natural history, which is the occurrence of either acute life-threatening illness in early infancy or unexplained developmental delay with intercurrent episodes of metabolic decompensation in later childhood. The incidence of individual inborn errors of organic acid and fatty acid metabolism varies from 1 in 10,000 to less than 1 in 1,000,000 live births, however, their collective incidence approximates 1 in 4,300 live births [14]. This estimation does not include other inborn errors of metabolism (i.e., amino acid disorders, urea cycle disorders, congenital lactic acidemias) for which diagnosis and monitoring may also utilize organic acid analysis.
A situation of severe and persistent metabolic acidosis of unexplained origin, elevated anion gap, and severe neurologic manifestations should always be considered as a strong diagnostic indicator for one of these diseases, findings that warrant immediate verification and should not be postponed, as occurs frequently, until more common causes have been ruled out. The presence of ketonuria, occasionally massive, provides an important clue toward the recognition of disorders such as methylmalonic aciduria, propionic aciduria, and isovaleric aciduria, particularly in the neonatal period, when physiologic ketosis is not seen. Hyperammonemia, hypoglycemia, and hyperlactic acidemia are frequently associated findings, particularly during acute episodes of metabolic decompensation. Plasma amino acid analysis may provide only limited and generally non-specific information for enzyme deficiencies affecting distal steps of amino acid catabolism. Therefore, the biochemical diagnosis of individual organic acidemias ultimately relies on urine organic acid analysis by gas chromatography/mass spectrometry (GC/MS). Detection, positive identification, and eventually quantification of pathognomonic organic acids by GC/MS could be available in a matter of hours if the analysis is performed at a local laboratory. Otherwise, results from a referral laboratory should be available within 24 hours for an urgent case. Direct communication with the laboratory director when an urgent sample is sent will help to expedite the diagnosis.
A large proportion of organic acidurias known to date affect the intermediate metabolism of the essential branched chain amino acids isoleucine, leucine, and valine. The diagnostic specificity of organic acid analysis under acute and asymptomatic conditions is outlined for individual defects in Table 24.5. Informative profiles may not always be detected in disorders where the excretion of diagnostic metabolites depends on the residual activity of the defective enzyme, the dietary load of precursors, or the anabolic status of a patient. In some cases, methods with higher specificity and sensitivity, such as acylcarnitine determination by electrospray ionization-tandem MS [15] and acylglycine determination by GC/MS stable isotope dilution analysis [16], can overcome the limitations of standard organic acid analysis to assess patients who are not acutely ill. However, these tests are rarely needed to diagnose the most frequent and acute organic acidurias.
Table 24.5 Organic acidurias: diagnostic significance of organic acid, acylcarnitine, and acylglycine analysisa
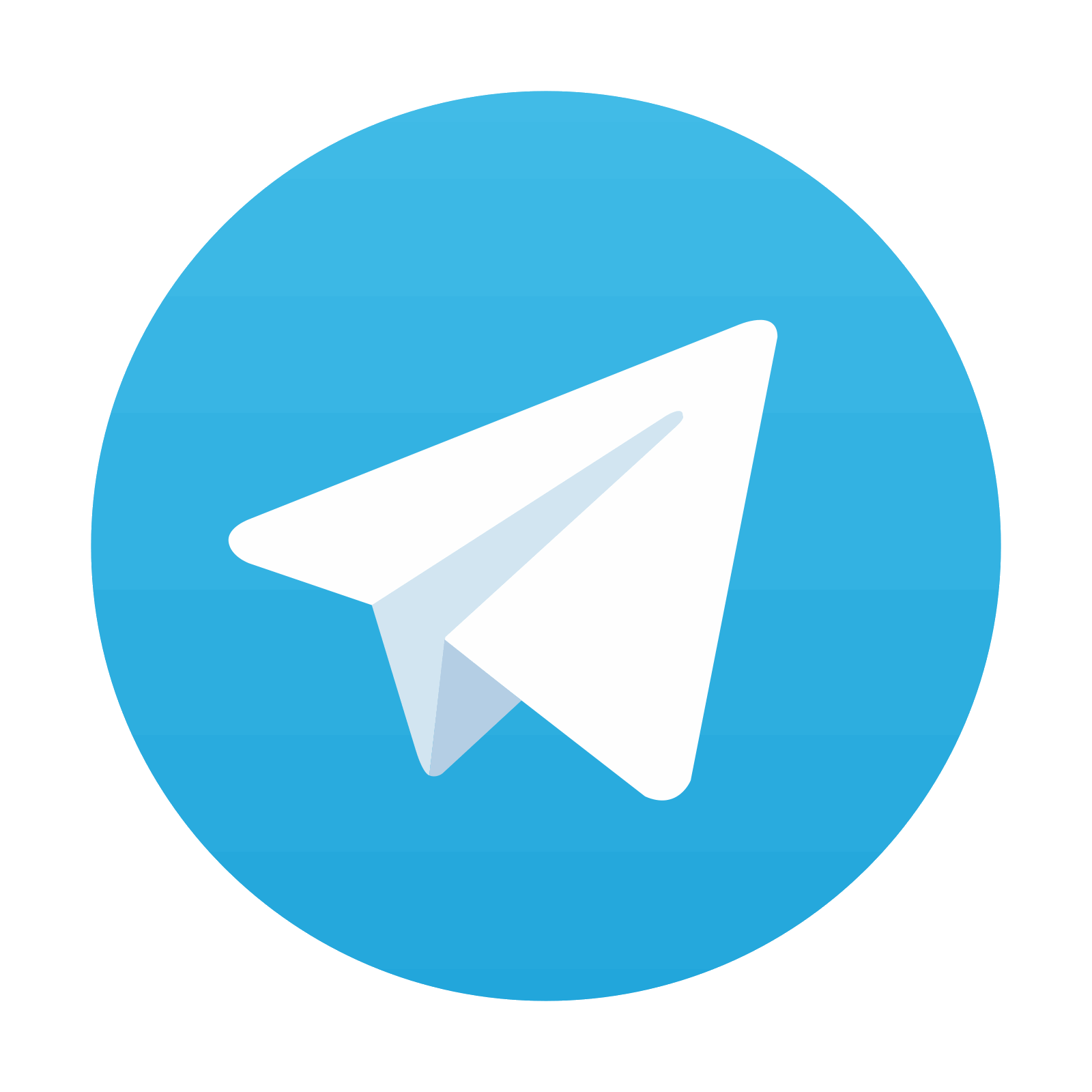
Stay updated, free articles. Join our Telegram channel
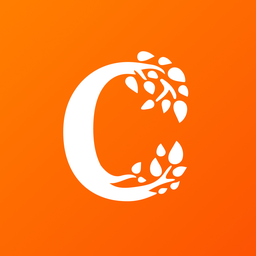
Full access? Get Clinical Tree
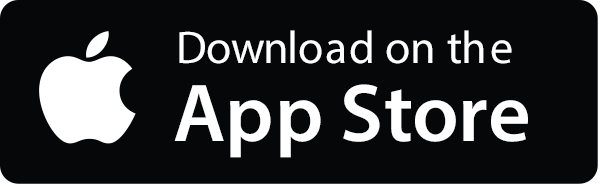
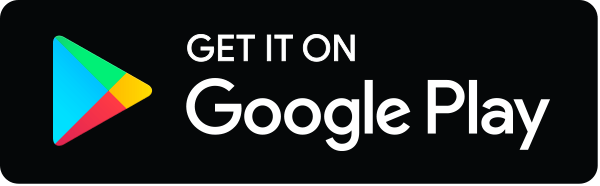
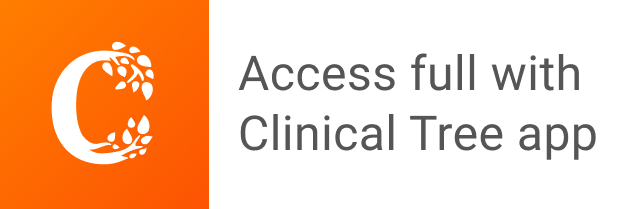