Abstract
The liver attains its highest relative size at about 10% of fetal weight at the ninth week of gestation. Early in gestation the liver is the primary site for hematopoiesis. At seven weeks of gestation, hematopoietic cells outnumber hepatocytes. Primitive hepatocytes are smaller than mature cells and are deficient in glycogen. As the fetus nears term, hepatocytes predominate and enlarge with expansion of the endoplasmic reticulum and accumulation of glycogen. Hepatic blood flow, plasma protein binding, and intrinsic clearance by the liver (reflected in the maximal enzymatic and transport capacity of the liver) also undergo significant postnatal maturation. These changes correlate with an increased capacity for hepatic metabolism and detoxification. At birth, the liver constitutes about 4% of body weight compared with 2% in the adult. Liver weight doubles by 12 months of age and increases three-fold by three years of age.
Introduction
The liver attains its highest relative size at about 10% of fetal weight at the ninth week of gestation. Early in gestation the liver is the primary site for hematopoiesis. At seven weeks of gestation, hematopoietic cells outnumber hepatocytes. Primitive hepatocytes are smaller than mature cells and are deficient in glycogen. As the fetus nears term, hepatocytes predominate and enlarge with expansion of the endoplasmic reticulum and accumulation of glycogen. Hepatic blood flow, plasma protein binding, and intrinsic clearance by the liver (reflected in the maximal enzymatic and transport capacity of the liver) also undergo significant postnatal maturation. These changes correlate with an increased capacity for hepatic metabolism and detoxification. At birth, the liver constitutes about 4% of body weight compared with 2% in the adult. Liver weight doubles by 12 months of age and increases three-fold by three years of age.
The functional development of the liver that occurs in concert with growth requires a complicated orchestration of changes in hepatic enzymes and metabolic pathways that result in the mature capacity of the liver to undertake metabolism, biotransformation, and vectorial transport. Greengard has established a paradigm for hepatic development based on a group of several hepatic enzymes studied in the rat and less extensively in humans. In one pattern of hepatic development, enzymatic activity is high in a fetus and falls during postnatal development. Examples would include thymidine kinase and ornithine decarboxylase [1]. The activities of other enzymes are expressed initially during early fetal development and continue to increase progressively after birth. Examples include glutamate dehydrogenase, fructose-1,6-diphosphatase, and aspartate aminotransferase [1]. Another group of enzymes is expressed perinatally and continues to increase progressively after birth. These enzymes include phosphoenolpyruvate carboxykinase (PEPCK) and uridine 50-diphosphate glucuronyl-transferase (UGT). A final pattern of development occurs with enzymes that are expressed significantly after birth and peak at weaning, including alanine aminotransferase and alcohol dehydrogenase.
The stepwise appearance of new groups of enzymes during development may be related causally to sequential changes in the level of circulating hormones [1]. For example, total serum thyroxine and triiodothyronine levels of the human fetus undergo a sudden increase between the ninth and tenth weeks of gestation. Similarly, fetal plasma concentrations of cortisol and cortisone are as high by the third month of gestation as at term. There is also a sudden increase in plasma glucagon at birth, which may influence the expression of the neonatal cluster of enzymes in rat liver. The final steps of biochemical differentiation, including the synthesis of enzymes necessary to process a solid diet, occur just before weaning in the rat. A natural surge in cortisone and thyroxine at this time may be important in mediating this change.
With advances in cellular and molecular biology, mechanisms underlying these developmental changes have been found to be extremely complicated and regulated at transcriptional, translational, and post-translational levels. A complete discussion of this topic is beyond the scope of this review. Only selected examples of developmental changes in the functional capacity of the liver are considered, particularly those with relevance to understanding susceptibility to liver disease.
Hepatic Energy Metabolism in the Fetus and Neonate
There is increasing epidemiological and experimental evidence that perturbations of perinatal energy homeostasis including maternal diabetes, maternal obesity or malnutrition, and intrauterine growth retardation can predispose to obesity and metabolic syndrome later in life. Therefore, non-alcoholic fatty liver disease (NAFLD), the most common liver disorder in adults and children and a feature of metabolic syndrome, in part has its origin in the fetus. Recent findings indicate that epigenetic methylation of critical genes involved in metabolism detected in human fetal tissues at birth is strongly associated with childhood total and central body adiposity, factors repeatedly found in patients with NAFLD [2].
Carbohydrate Metabolism
Glucose is the primary fuel for the fetus and accounts for 50–80% of energy consumption. The fetus is completely dependent upon the mother for the continuous transfer of glucose across the placenta [3]. Maternal glucose production increases by approximately 15–30% in late gestation. The availability of glucose to the fetus is also enhanced by maternal insulin resistance. At birth the neonate must rapidly transition to independent control of glucose homeostasis. The tenuous nature of perinatal–neonatal glucose metabolism is exemplified by the multiplicity of disorders associated with neonatal hypoglycemia, including many liver diseases [3].
Glycogen synthesis begins in the fetus at about the ninth week of gestation, with glycogen stores rapidly accumulating near term, at which time the fetal liver contains an amount of glycogen two to three times higher than that in the adult liver (40–60 mg/g liver) [3]. Endometrial stromal cells (EnSCs) accumulate and secrete a variety of nutritive molecules that are absorbed by trophoblastic cells and transmitted to the fetus. The hormone adiponectin controls glycogen metabolism and trafficking in these cells, thus facilitating transfer of energy to the fetus [4].
These large stores of hepatic glycogen are important for maintenance of blood glucose levels during the perinatal period before other energy sources are available and before the onset of hepatic gluconeogenesis. Since efficient regulation of the synthesis, storage, and degradation of glycogen develops only near the end of a full-term gestation, there is a propensity to hypoglycemia in preterm infants. Other sources of carbohydrates, particularly galactose, are converted to glucose, but there is substantial dependence on glucogenesis for supplies of glucose early in life, particularly if glycogen stores are low. There is significant re-accumulation of glycogen around the second postnatal week and stores reach adult levels at about three weeks in normal full-term infants [3]. The blood glucose concentration in the neonate can be maintained for about a 10- to 12-hour fast by glycogenolysis until hepatic glycogen is reduced to <12 mg/g liver. The mechanisms underlying the initiation of hepatic glycogenolysis postnatally are not defined completely, but a number of features are known to occur:
1. Accumulation of glycogen in fetal liver (to levels which are two- to three-fold higher than in the adult by term).
2. Low rates of gluconeogenesis by fetal liver.
3. Low rates of glucose use by fetal liver.
4. Amino acids form an important energy source for fetal liver (extensive transamination and oxidative degradation).
5. High capacity of fetal liver for fatty acid synthesis.
6. Rapid induction of ability to oxidize fatty acids during the first days of life.
7. Fatty acid oxidation which is critical to support hepatic gluconeogenesis.
8. Rapid increase in hepatic ketogenesis after birth.
Another important feature of fetal hepatic carbohydrate metabolism is a deficiency of glucose-6-phosphatase activity. This enzyme, which is present in the liver and kidney, is a microsomal enzyme that is involved in the last step of hepatic glucose synthesis [3]. Gluconeogenic flux is directed into hexose and pentose phosphate pools and to glycogen formation, with minimal fetal glucose production in the liver. There is evidence that the hormone adiponectin is involved in feeding the fetus during the first trimester by controlling glycogen metabolism in both the uterus and the placenta [4]. The level of glucose-6-phosphatase increases to near adult levels at term and rises further after birth. Gluconeogenesis, the synthesis of glucose from lactate, amino acids, and other small molecules, does not occur at significant rates in the fetal liver [3]. Fetuses are hyperinsulinemic, and insulin is known to behave as an inhibitor of the gluconeogenic gene expression program. Maternal insulin stimulates placental glucose transport by promoting trafficking of glucose transporters to the placental basal plasma membrane, thus enhancing glucose transfer to the fetus [5]. In animal studies, fetal glucose utilization has been shown to be approximately equal to umbilical glucose uptake over a wide variety of maternal glucose concentrations. The enzymes necessary for hepatic gluconeogenesis are present in the near-term fetus. However, the level of activity of the rate-limiting enzyme of gluconeogenesis, PEPCK, is extremely low in the late-gestation fetus and increases rapidly after birth [3].
Changes in several other hepatic enzymes underlie differences in carbohydrate metabolism between the fetus and neonate [5]. For example, there is a deficiency of hepatic glucokinase, a high Km glucose-phosphorylating enzyme, until the time of weaning. In contrast, the amount of activity of hexokinase I, a low Km glucose-phosphorylating enzyme, is high in fetal liver and declines at the end of gestation. Hepatic glucose uptake may be limited by the ability to phosphorylate glucose in the fetal and neonatal liver. The activity of hepatic galactokinase, the enzyme that phosphorylates galactose, the other major hexose in the neonatal diet, rapidly increases near term, probably to assimilate the large intake of galactose in the newborn diet. Glucose may be taken up by the fetal liver, but this process is inhibited by lactate, amino acids, and fatty acids and is not stimulated by insulin as it is postnatally. Glucose utilization by the fetal liver is low, owing to the use of alternative fuels such as amino acids and lactate. A mechanism possibly available to increase hepatic glucose uptake is an increase in glycolytic flux resulting from a decrease in the hepatic concentration of glucose 6-phosphate [3]. The levels of activity of several key enzymes that can decrease glucose 6-phosphate, including glucokinase and pyruvate kinase, are low in fetal liver, impairing the ability of the fetal liver to increase glucose uptake by decreasing the hepatic concentration of glucose 6-phosphate.
There appears to be little hepatic glucose uptake in the neonate [5]. Animal studies have shown preferential hepatic uptake of galactose and lactate after a meal, with incorporation of galactose into glycogen or its conversion to glucose. Glucose appears to be delivered for use by peripheral tissues; galactose is used preferentially by the liver for carbohydrate synthesis.
After birth and before the onset of suckling there is a time lapse in which the newborn undergoes a unique kind of starvation [3]. During this period, glucose is scarce and ketone bodies are not available, because of the delay in ketogenesis. Under these circumstances, the newborn is supplied with another metabolic fuel, lactate, which is utilized as a source of energy and carbon skeletons [3]. Neonatal rat lung, heart, liver, and brain utilize lactate for energy production and lipogenesis. Recent studies using stable isotopes have shown that gluconeogenesis from lactate and pyruvate is established by four-to-six hours after birth. Gluconeogenesis from pyruvate contributes as much as 30% to total glucose production in healthy term babies between five and six hours after a feed. Both glycogenolysis and gluconeogenesis are stimulated by the surges of serum catecholamines and glucagon associated with birth.
After the initiation of suckling, plasma insulin levels fall and glucagon and catecholamines rise. Theses hormones activate hepatic glycogen phosphorylase, which induces glycogenolysis, maintaining glucose levels immediately after birth [3]. When liver stores of glycogen are exhausted after 12 hours, gluconeogenesis is then required. The low blood glucose levels and increased cortisol levels activate hepatic glucose 6-phosphatase, and PEPCK is induced by the reversal of the insulin/glucagon ratio. Together these adaptations lead to increased hepatic glucose release from gluconeogenesis. The molecular mechanisms underlying this dramatic change have not been completely elucidated, but PEPCK, which catalyzes the initial step in hepatic gluconeogenesis, is tightly regulated by glucagon, glucocorticoids, thyroid hormone, insulin, and glucose. A number of transcription factors that bind to the PEPCK gene promoter appear to be important in the process, including the glucocorticoid receptor, the retinoic acid receptor, the retinoid X receptor, the forkhead box family members, CCAAT/enhancer binding protein (C/EBPα), cAMP response element binding protein (CREB), COUP transcription factor 2, and hepatocyte nuclear factor (HNF) 4α [6]. The peroxisome proliferator-activated receptor-coactivator 1α (PGC1α) is a transcriptional coactivator thought to be a master regulator of liver energy metabolism through its capacity to activate genes involved in gluconeogenesis, including those for HNF4α and GR. Another transcription factor, FOXO1, has also been shown to be required for the gluconeogenic action of PGC1α. However, PGC1α ̣is expressed at much higher levels iṇ rat fetal than adult liver. After birth, PGC1α is not induced further unless the animals are fasted, even as gluconeogenesis is markedly induced. It is unknown why PGC1α seems to be partially disassociated from regulation of gluconeogenesis in the fetus and newborn [6]. These data give some sense of the complexity of the changes that occur in gene expression that allow maintenance of blood glucose concentrations after birth.
Amino Acid Metabolism
The fetus is highly dependent on the influx of amino acids across the placenta [7, 8]. System A amino acid transporters in the syncytiotrophoblast microvillous plasma membrane are directed toward maternal blood, actively accumulating amino acids, while system L transporters mediate uptake of essential amino acids from the maternal circulation. The functional capacity and protein abundance of these transporters in the placenta are critical to fetal growth. There are many maternal nutritional and endocrine signals regulating placental amino acid transport including insulin, insulin-like growth factors, adipokines, and steroid hormones in conjunction with growth signals originating from the fetus. There is a high rate of hepatic uptake of all of the essential and most of the non-essential amino acids by the fetal liver including all of the gluconeogenic amino acids. In the fetus, the carbon from these amino acids is released primarily as glutamate and pyruvate with a smaller amount of hepatic release of serine, ornithine, and aspartate [7]. In rodent studies, the uptake of glutamine, alanine, and lysine by the liver is much greater than their incorporation into protein. The large uptake of amino acids by the liver and the increase in hepatic concentrations of free amino acids during gestation, which decline after birth, likely contribute to the synthesis of other substrates, such as glycogen and glucose. Interorgan cycling between the fetal liver and placenta has been proposed for non-essential amino acids like glycine and serine.
Most of the enzymes required for regulation of amino acid metabolism are expressed at birth. However, there may be delayed appearance of the activity of p-hydroxyphenylpyruvate oxidase, a key enzyme in the degradation of tyrosine. A relative deficiency of this enzyme is thought to cause transient neonatal tyrosinemia.
The use of amino acids by the fetal liver may differ significantly from that by the adult [8]. For example, there is preferential use of the β-carbon of serine for DNA synthesis by fetal liver and for RNA synthesis by adult liver. The high concentration of free amino acids in fetal liver may have a key role in regulation of hepatic growth. For example, high concentrations of free amino acids suppress intralysosomal proteolysis. Many amino acids are transported actively by the placenta through specific carrier mechanisms. Net flux of amino acids from placenta to fetus has been demonstrated for all essential amino acids and most non-essential amino acids except for aspartate, glutamate, and serine. Most amino acids also are taken up avidly by the fetal liver [8]. There is evidence for net production of serine, glutamate, and aspartate by the fetal liver, with no umbilical uptake. Studies in the pregnant sheep model indicate that maternal serine is not transferred to the fetus but is metabolized, in large part to glycine, which is transferred to the fetus and taken up by the fetal liver [8]. Fetal serine requirements are largely met by production in the liver via the action of serine hydroxymethyltransferase and the glycine cleavage system. The uptake of some neutral and basic amino acids by the placenta is in considerable excess of the estimated growth requirements, providing further evidence that some amino acids undergo extensive transamination and oxidative degradation in the fetus.
Human fetal amino acid metabolism was recently studied in healthy pregnant women before elective cesarean section at term, using continuous stable isotope infusions of the essential amino acids [9]. Fetuses showed significant leucine, valine, and methionine uptake and turnover rates. There was net transport of α-keto-isocaproate, but not α-keto-isovalerate (the leucine and valine ketoacids, respectively) from the fetus to the placenta. The data suggested high oxidation rates of leucine and valine, up to half of net uptake. The results are consistent with high rates of protein breakdown and synthesis, comparable with, or even slightly higher than, that in premature infants. The relatively large uptakes of total leucine and valine carbon also suggest high fetal oxidation rates of these essential branched-chain amino acids.
The hepatic uptake of glutamine in the fetus appears to be greater than that of any other amino acid [10]. The placenta is a major source of the glutamine that enters the fetal circulation. Net flux of glutamine from the maternal circulation into the placenta, large in comparison to the net fluxes of other amino acids, occurs and is augmented by placental glutamine synthesis. After glutamine transport into the fetal circulation, the fetal liver is the primary site for its uptake and for glutamate production and, as such, determines the glutamate supply to the placenta [10]. Glutamine is converted to glutamine and ammonia by glutaminase. The placenta can also produce glutamate by branched-chain amino acid transamination. Although glutamine is a gluconeogenic amino acid, its uptake by the fetal liver is not coupled with a significant amount of fetal hepatic gluconeogenesis. The fetal liver releases glutamate, which is taken up by the placenta and for the most part rapidly oxidized. A significant proportion of the remaining glutamine that is not metabolized to glutamate in the liver and transported to the placenta is used by the fetal tissues for growth.
The distribution and zonation of enzymes involved in glutamine metabolism during development have been evaluated extensively [10]. Glutamine synthetase catalyzes the reaction of glutamate and α-ketoglutarate with the production of glutamine. This ammonia-scavenging pathway has been studied in developing rodent liver. In adult liver, glutamine synthetase protein and mRNA are localized exclusively to the hepatocytes immediately adjacent to central veins. This restricted localization correlates with hepatic uptake of metabolic precursors used by glutamate synthetase, such as glutamate and α-ketoglutarate. Ornithine aminotransferase is colocalized to this area also. In contrast, in fetal mouse liver, glutamine synthetase mRNA is expressed in all fetal hepatocytes at mid-gestation and at term, but the enzyme protein is not detectable [11]. The shift to the adult pattern of glutamine synthetase localization in the perivenous hepatocytes occurs postnatally. This mature pattern of perivenous expression is a permanent feature of the adult liver. There is no regression to the fetal pattern with liver injury or with regeneration.
The capacity for urea synthesis by the fetal liver is well established by mid-gestation, and the liver serves as the major site for ammonia clearance in the fetus. There is significant endogenous fetal ammonia production by peripheral tissues, with hepatic clearance. Owing to a mature complement of urea cycle enzymes by mid to late gestation, there is a capacity to increase urea production with increased ammonia and nitrogen uptake.
The transulfuration pathway is an aspect of amino acid metabolism that has been well studied and has important implications for nutrition of the infant. A low level of cystathionase activity in the fetus impairs the transsulfuration pathway by which dietary methionine is converted to cysteine. After birth there is a rapid increase in cystathionase activity in the human liver. Moreover, stable isotope studies in term human neonates confirm robust transsulfuration of methionine, evidencing that cysteine is not a conditionally essential amino acid in the neonatal period [12]. The level of cysteine is 50% higher than that of methionine in human milk, supporting the notion that a sufficient quantity of this amino acid must be provided to the neonate. Similar dietary requirements may exist for other sulfur-containing amino acids, such as taurine.
Taurine is considered a “conditionally” essential amino acid, particularly in the neonate, and is not incorporated into proteins [13]. Although taurine accounts for only 3% of the free amino acid pool in plasma, it accounts for 25% of this pool in liver. In addition to its defined role in the bile salt conjugation, taurine is also involved in membrane stabilization, osmoregulation, modulation of calcium flux, antioxidation, neuromodulation, cell proliferation, and immune regulation. Cysteine sulfinic acid decarboxylase is a rate-limiting enzyme for taurine biosynthesis in the human. Another key enzyme for cysteine metabolism, cysteine dioxygenase, has a critical role in determining the flux of cysteine between cysteine catabolism/taurine synthesis and glutathione synthesis. Preterm infants are particularly dependent on an adequate dietary intake to maintain plasma taurine levels because renal immaturity impairs tubular reabsorption and an immature enzymatic pathway limits biosynthesis.
Lipid Metabolism
After the first third of gestation, enhanced lipolytic activity in maternal adipose tissue related to estrogen stimulation and insulin resistance contributes to hyperlipidemia; there is an increase in plasma triacylglycerol concentrations as well as smaller rises in phospholipid and cholesterol concentrations [14]. The catabolic state of pregnancy favors maternal tissue lipid use as energy sources, thus sparing glucose and amino acids for the fetus. Maternal hypertriglyceridemia contributes to fetal growth and development and serves as an energy depot for maternal dietary fatty acids [15]. Triglycerides do not cross the placental barrier, but the presence of lipoprotein receptors in the placenta, along with lipoprotein lipase, phospholipase A2, and intracellular lipase activities, lead to the release to the fetus of polyunsaturated fatty acids transported as triglycerides in maternal plasma lipoproteins [16].
Maternal cholesterol is essential for fetal use as components of cell membranes and as a precursor of bile acids and steroid hormones. Although the fetus relies on maternal cholesterol during early gestation, during late pregnancy fetal tissues have a high capacity to synthesize cholesterol [15].
Free fatty acids oxidized in the maternal liver as ketone bodies provide an alternative fuel for the fetus, augmenting glycogenolysis and gluconeogenesis [16]. The fetus obtains fatty acids through de novo synthesis, passive diffusion of non-esterified fatty acids across the placenta, and selective maternofetal placental transport for certain fatty acids, particularly physiologically important long-chain, polyunsaturated fatty acids [15]. The modest amounts of free fatty acids transferred across the placenta are stored in the liver and adipose tissue and are not used by peripheral tissues. The capacity for fatty acid synthesis by the fetal liver is high, peaking in mid-gestation [17]. In rodent models, the level of acetyl CoA carboxylase, the rate-limiting enzyme for fatty acid synthesis in adult liver, is low, suggesting an alternative mechanism for provision of CoA derivatives [15]. Maternally derived ketones and glucose may be precursors for fatty acid synthesis by the fetal liver [15].
Although some fatty acids can passively diffuse across the placenta, much less is known about transport of other lipids. Recent studies have found that mRNAs for apolipoprotein B (apoB) and microsomal triglyceride transfer protein are expressed in the human placenta [18]. Term placental tissue was found to produce and secrete apoB-100 particles in vitro. The apoB-containing lipoproteins carry cholesterol and triglyceride as well as fat-soluble vitamins and glycoproteins. The term placenta weighs approximately four times more than the fetal liver so is likely to make a significant contribution to the fetal plasma pool of apoB-containing lipoproteins.
Fat that accumulates in the fetal liver is mobilized soon after birth, not for export of free fatty acids but for local utilization [18]. The oxidation of fat results in significant generation of adenosine triphosphate (ATP) for energy and ketone body formation for use by peripheral tissue [16]. Similar to adult liver, a lysosomal acid lipase mediates the breakdown of triacylglycerols. There is rapid maturation of the ability of the liver to oxidize fatty acids during the first days of life. The liver is the main source for synthesis of ketone bodies used by other tissues. The concentrations of ketone bodies, including acetoacetate, 3-hydroxybutyrate, and acetone, increase in the blood during the first 24 hours after birth. In the liver, the postnatal development of long-chain fatty acid oxidation and ketogenesis is regulated by pancreatic hormones, the levels of which change markedly at birth, with a fall in insulin and a rise in glucagon levels.
The postnatal increase in hepatic fatty acid oxidation is critically important in supporting hepatic gluconeogenesis. Hepatic fatty acid β-oxidation is activated after birth to produce energy from breast milk lipids. The nuclear receptor PPARα-dependent DNA demethylation leads to up-regulation of the hepatic fatty acid β-oxidation genes during the neonatal period [19].
Milk feedings provide the major source for calories postnatally; this high-fat, low-carbohydrate diet supports active gluconeogenesis to maintain levels of blood glucose [17]. Both long- and medium-chain fatty acids from the diet stimulate gluconeogenesis by increasing the hepatic supply of gluconeogenic precursors and activating hepatic gluconeogenesis. In rodent models, the rate of hepatic lipogenesis decreases just prior to birth, as a result of a reduction in the activity of lipogenic enzymes. The large amounts of non-esterified free fatty acids present during the suckling period may underlie the inhibition of acetyl CoA carboxylase activity. At weaning, the lipogenic capacity of the liver increases in response to a high-carbohydrate diet. The specific activity and amount of mRNA for fatty acid synthase and acetyl CoA carboxylase increase significantly in the liver [18].
There is a marked increase in plasma free fatty acid concentrations after birth in infants [14]. Fatty acids varying in their chain length and degree of saturation have very specific roles in metabolism. Short-chain fatty acids may act as local growth factors in the intestine; medium and saturated long chain fatty acids are important sources of energy; polysaturated long-chain fatty acids are involved in metabolic regulation; and very-long-chain fatty acids are important structural components of membranes [18]. Free fatty acids may supply approximately 42 kJ/kg daily of energy for the newborn. Fat stores complementing gluconeogenesis in a newborn may be particularly important for the infant who is small for gestational age [20]. The induction of fatty acid oxidation that occurs at birth is part of a coordinate increase in hepatic gluconeogenesis that occurs after birth in an effort to adapt to an alteration in energy supply.
Biotransformation
The liver is the main site for metabolism of drugs and xenobiotics, and therefore is unusually susceptible to structural and functional injury following exposure to drugs and toxins. Infants and children may be more or less vulnerable to toxic liver injury than adults. Immaturity of pathways for biotransformation may prevent efficient degradation and elimination of a toxic compound; in other circumstances, the same immaturity may limit the formation of a reactive metabolite [21].
Many variables influence drug metabolism, including liver size, liver blood flow, plasma protein binding, and intrinsic clearance (a product of the enzymatic and transport capacity of the liver) [21]. Infants and young children actually have a greater liver-to-body mass ratio than adults, but many of the functions of the hepatocyte involving detoxification, energy metabolism, and excretion of waste require significant pre- and postnatal maturation to attain adult capacity. The underlying pharmacogenetics may also influence either the affinity or the capacity of an enzyme responsible for biotransformation of a drug or toxin. The development of the array of mechanisms involved in the process for the most part is not completely defined [22].
Three general stages of drug metabolism occur in the liver: phase I reactions (oxidation, reduction, and hydrolysis), phase II reactions (synthetic conjugations with sulfate, acetate, glucuronic acid, glycine, and glutathione), and phase III processes (export out of the liver via transporters on the sinusoidal and canalicular membranes) [22]. Many phase I and phase II enzymes that are critically important for drug metabolism are polymorphically expressed, developmentally regulated, and subject to considerable inducibility because of exposure to drugs, xenobiotics, and environmental factors. This has a number of effects:
1. Decreased capacity of the neonatal liver to metabolize, detoxify, and excrete many drugs.
2. Deficiency of many enzymes required for oxidative, reductive, hydrolytic, and conjugation reactions.
3. Early production of many cytochrome P450 (CYP) enzymes in the embryo and fetus, such as CYP3A7, which is involved in steroid metabolism.
4. Delayed expression of other CYP enzymes, such as CYP1A2, important in drug metabolism.
5. Reduced activity of many phase II enzymes, including uridine diphosphate glucuronyltransferases in the fetus and neonate.
The xenobiotic nuclear receptors (NRs), the pregnane X receptor (PXR, also known as the steroid and xenobiotic receptor), the constitutive androstane receptor (CAR), and the aryl hydrocarbon receptor (AhR) coordinately induce genes involved in the three phases of xenobiotic metabolism, including oxidative metabolism, conjugation, and transport [23]. Many xenobiotics are ligands for the orphan NRs, CAR and PXR, which both heterodimerize with the retinoid X receptor (RXR) and transcriptionally activate the promoters of many genes involved in drug metabolism. Similarly, the AhR, which dimerizes with the AhR nuclear translocator in response to many polycyclic aromatic hydrocarbons, regulates genes for the CYP enzymes. Immunoreactivity for AhR can be detected in human fetal liver, but there is little else known about its development. Both PXR and CAR are expressed at low (and highly variable) levels in pre- and neonatal human liver relative to liver tissue derived from older children, with CAR expressed at higher levels relative to PXR in prenatal liver. In contrast, mRNA expression of the heterodimer partner RXRα was less variable and did not differ appreciably between pre- and postnatal liver samples. Information is lacking about expression of the many coactivators and histone-modifying enzymes that are attracted to the gene promoters upon ligand binding and needed for the activity of NRs.
The CYP enzymes perform the majority of phase I reactions. In humans, approximately 59 CYP enzymes have been identified and these are classified into families according to sequence homology [24]. Overall levels are highest in the liver, but significant and even exclusive expression can be found for some CYPs in other tissues. Developmental expression of CYP enzymes is one of the key factors determining the pharmacokinetic status pre- and postnatally [21]. Drug-metabolizing CYP enzymes, the major phase I enzymes, are active in human liver at very early stages of intrauterine development, albeit at much lower concentrations than in the adult. The liver of the human fetus and even the embryo possesses relatively well-developed metabolism of xenobiotics. For example, there is experimental evidence for the presence of CYP1A1, CYP1B1, CYP2C8, CYP2D6, CYP2E1, CYP3A4, CYP3A5, and CYP3A7 in the fetal liver after the embryonic phase (approximately eight to nine weeks of gestation) [25]. Significant xenobiotic metabolism also occurs during organogenesis (before eight weeks of gestation). CYP3A7 is the primary cytochrome P450 enzyme expressed in fetal liver, but declines rapidly after birth [21].The major increase in both activity and number of different enzymes takes place after birth, probably during the first year of life. Information concerning these developmental changes in the human is incomplete [25]. Figure 2.1 shows the postnatal development of several of the more important CYP450 enzymes.
CYP1A1 is also present during organogenesis and metabolizes exogenous toxins, some of which are procarcinogens [24]. Production of CYP1A1 declines with age and it is not detectable in adult liver. CYP1A2, important in its metabolism of caffeine and theophylline, is not produced significantly in fetal liver, exists at a very low level in the neonate, but reaches adult levels by the fourth or fifth postnatal month [25]. CYP1A2 was also not detectable in fetal liver samples (gestation 14 to 40 weeks). A progressive increase in CYP1A2 catalytic activity and protein levels has been measured: in neonatal samples at 4–5% of adult levels, in samples from infants aged 1–3 months at 10–15%, in samples from children aged 3–12 months at 20–25%, and in samples from children aged 1–9 years at 50–55% [25].
The CYP2C subfamily metabolizes many clinically important drugs: CYP2C9 is the major hepatic CYP2C enzyme, followed by CYP2C19 and CYP2C8, and these together are responsible for the oxidative metabolism of approximately one-third of clinically important drugs [25]. Both CYP2C8 and CYP2C9 are minimally expressed in fetal liver [24]. CYP2C9, which metabolizes phenytoin, achieves adult activity by one to six months postnatally and exceeds adult activity by three to ten years of age. Eleven polymorphisms of CYP2C9 have been detected and this adds a further layer of complexity in considering the therapeutic efficacy or potential for toxicity of drugs such as ibuprofen and indomethacin, which are used in the neonate and are substrates for this enzyme. Protein and catalytic activity for CYP2C19 that were 12–15% of mature values were observed as early as eight weeks of gestation and were similar throughout the prenatal period. CYP2C19 expression did not change at birth, increased linearly over the first five postnatal months, and varied 21-fold from five months to ten years. Adult CYP2C19 protein and activity values were observed in samples from children older than ten years [25].
CYP2E1 is present in some second-trimester fetuses and is involved in metabolism of organic solvents including alcohol and is the primary enzyme involved in the generation of N-acetyl-p-benzoquinone imine, the hepatotoxic metabolite of acetaminophen. CYP2E1 activity is low in the fetus, reaches 30–40% of adult levels by one year of age, and is fully expressed by ten years of age [21]. After birth, hepatic CYP2D6 becomes active. CYP2D6 has many genetic polymorphisms leading to differing capacities to metabolize exogenous drugs, including psychotropic drugs and antihypertensives [25].
The CYP3A subfamily is the most abundant of the CYP enzymes and is involved in the metabolism of approximately 50% of commonly used medications [21]. CYP3A7, the major fetal hepatic cytochrome (30–50% of total liver P450), is uniquely present during organogenesis and is involved in steroid metabolism. Expression declines after the first postnatal week and is undetectable in most livers by one year of age. Variably detectable in the fetus, CYP3A5 is expressed at significant levels in about half of all children [25]. CYP3A4 is the major functional member of the CYP3A subfamily expressed postnatally and metabolizes over 75 drugs. CYP3A4 expression is low in the fetus and newborn but reaches 50% of adult values between six and 12 months of age [25]. CYP3A4 is induced by many drugs, including phenytoin and rifampin, and can be inhibited by erythromycin, cimetidine, and many other commonly used agents.
The flavin-containing monooxygenases (FMOs), encoded by six genes, mediate the NADPH-dependent oxidative metabolism of a wide variety of drugs, such as chlorpromazine and promethazine, as well as environmental toxins [26]. The development of FMO isoforms 1, 2, 3, 4, and 5 has been studied in human tissues. A comparison between fetal liver and adult liver showed that FMO1 was the only FMO that was low; all other FMOs had greater amounts of mRNA than in adult liver; FMO5 was the most prominent FMO form detected in fetal liver.
The development of phase II enzymes, including glucuronosyl transferases, sulfotransferases (SULTs), glutathione S-transferases (GSTs), N-acetyltransferases (NATs), and methyl transferases, has been studied less well than the CYP system. Sufficient information is available to indicate that important differences in the activities of these enzymes exist between children and adults and that each phase II enzyme for which data are known follows a distinct pattern of development.
An important group of conjugation reactions are catalyzed by the UGTs. There are more than ten known isoforms; these are involved not only in the glucuronidation of many hydrophobic drugs such as morphine and acetaminophen but also in the biotransformation of important endogenous substrates, including bilirubin and ethinylestradiol. However, isoform specificity for these substrates has not been fully characterized. Serious adverse events associated with inadequate glucuronidation of chloramphenicol in the neonate have highlighted the importance of developmental changes in UGT activity [24]. There are significant isoform-specific differences, which preclude a generalization of a simple developmental pattern for UGT activity. UGT2B7 is the only UGT isoform that has been characterized during ontogeny, both in vitro and in vivo, using morphine as the probe drug [25]. Glucuronidation of morphine in fetuses aged 15–27 weeks is only 10–20% that of the adult. Morphine metabolism usually reaches adult capacity between two and six months after birth but may not fully mature in some individuals until 30 months of age. Genetic polymorphisms have been identified for the UGT family, not only affecting UGT1A, which glucuronidates bilirubin, but also three other UGT isoforms. Mutations of UGT1A lead to Crigler–Najjar and Gilbert syndromes, inherited forms of hyperbilirubinemia. The impact of these genetic differences on drug metabolism remains to be established because of overlapping isoform specificity of the drugs studied as well as a lack of specific probe substrates to test the activity of individual UGT isoforms in relation to these mutations.
The GST family is a group of dimeric enzymes that conjugate glutathione to a wide variety of electrophilic compounds. The eight different GST classes demonstrate considerable overlap in substrate specificity [27]. The developmental expression of these enzymes is not well defined. Hepatic GSTA1 and GSTA2 have been detected at ten weeks of gestation and attain adult levels by one to two years of age [28]. Although GSTM, encoding GSTμ, is minimally expressed in the fetus, it dramatically increases to adult levels after birth. In contrast, GSTP1 is highly expressed in the first trimester and decreases progressively through gestation. Enzyme activity is still detectable in the neonate but is absent from adult liver. The functional significance of these ontogenic changes remains unknown.
The development of NAT2 has been studied in human fetal liver. Genetic variation in the NAT2 locus accounts for the rapid or slow acetylator status of individuals that is important in metabolism of isoniazid. Acetylation activity by this enzyme was absent during the first 14 weeks of gestation, with some activity detected by 16 weeks. All infants between 0 and 55 days of age were phenotypically slow acetylators, whereas 50% of infants aged 122–224 days and 62% of those aged 225–342 days were found to be fast acetylators. Activity of NAT2 seemed to be fully determined by three years of age, with 50% expressing the slow acetylation phenotypes, similar to the adult population [29].
The SULT family catalyze the transfer of a sulfuryl group to a plethora of drugs, endogenous substrates, and xenobiotics. There are at least 11 isoforms with overlapping substrate specificity. The pattern and extent of expression of the various SULT isoforms during development are not well defined [24]. Several isoforms have been studied in detail. Hepatic SULT2A1, important for steroid metabolism, is at low levels at 25 weeks of gestation and increases to near adult levels in the neonate. Hepatic SULT1A3 is involved in catecholamine metabolism and is highly expressed in early gestation and progressively declines through the late fetal and neonatal periods. The enzyme cannot be detected in adult liver. SULT1E1, a cardinal estrogen-inactivating enzyme, achieved the highest levels of expression during the earliest periods of gestation in prenatal male livers, indicating a requisite role for estrogen inactivation in the developing male. Overall, fetal and neonatal livers have significant capacity for sulfation at a time when other phase II enzymes critical for detoxification, particularly UGTs, are poorly developed.
Epoxide hydroxylase is an enzyme critical to the hydrolysis of epoxide metabolites produced by phase I reactions [25]. Microsomal (EPHX1) and cytosolic (EPHX2) forms exist. Substrates for microsomal epoxide hydroxolase include arene oxide intermediates of several aromatic anticonvulsants, such as phenytoin and carbamazepine. In human fetal liver, microsomal epoxide hydroxylase activity has been found to be correlated weakly with both increasing gestational age and protein concentration. After 22 weeks of gestation, fetal activity is approximately 50% of that observed in adult liver. There is less known about the ontogeny of the cytosolic form. Enzyme activity can be detected in fetal liver at 14 weeks of gestation and by 27 weeks is about 20% of activity in adult liver [25].
There is limited information about the development of membrane transport proteins that participate in phase III of drug metabolism including uptake into hepatocytes and excretion into bile [30]. The multidrug resistance gene 1 (MDR1) encodes a critical efflux pump, P-glycoprotein, located on the canalicular membrane of hepatocytes. MDR1 mRNA is very low in human fetal and neonatal livers in comparison with young adults. The multidrug resistance-related protein 2 (MRP2, gene ABCC2) is an ATP-dependent transport protein mediating the excretion of multivalent anionic molecules including glutathione conjugates and glucuronidated compounds. The mRNA for MRP2 has recently been studied in human fetal livers at gestational age 14–20 weeks and was present at 50% of the amount found in adult livers. Moreover, MRP2 showed mainly canalicular localization, which was indistinct compared with adult liver [31].
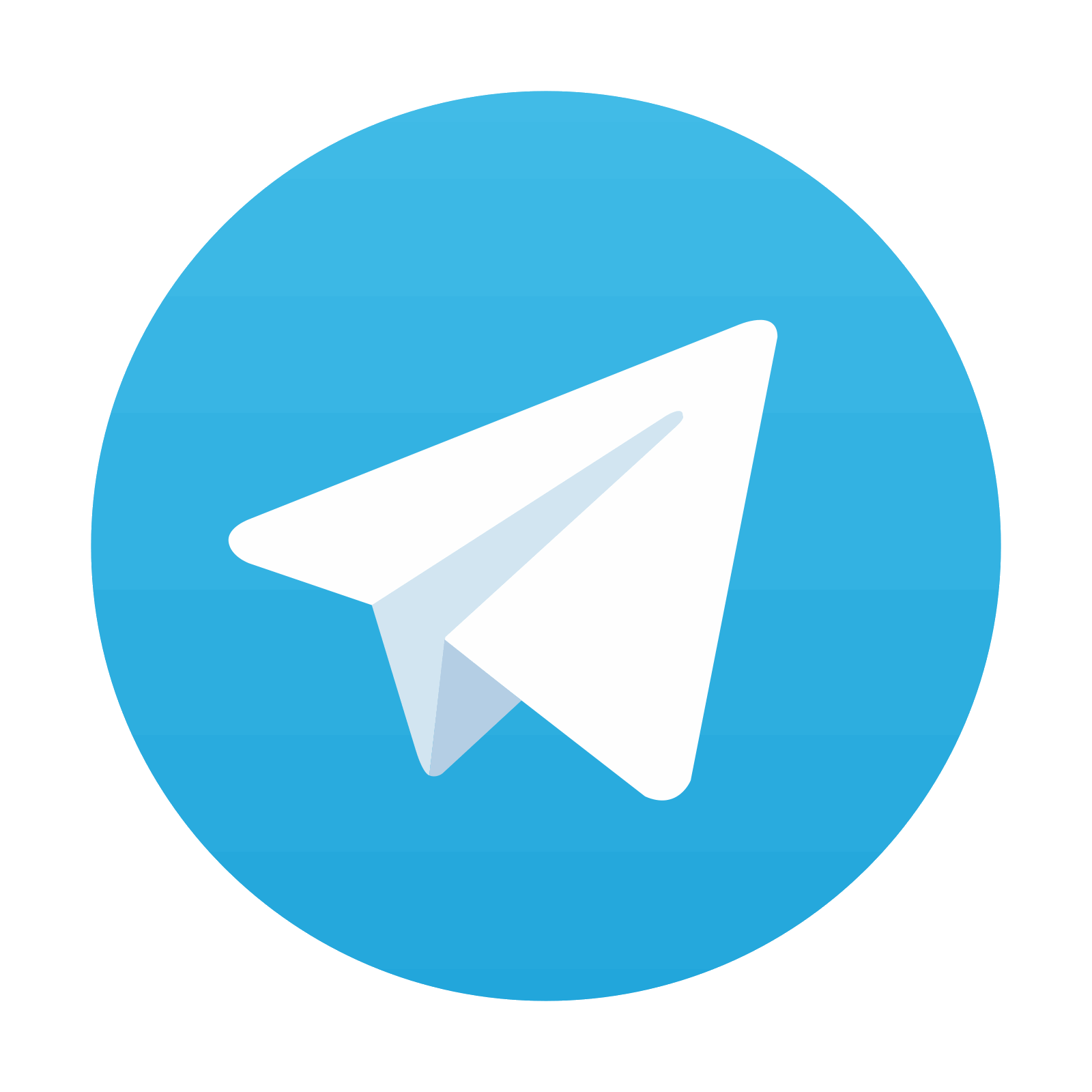
Stay updated, free articles. Join our Telegram channel
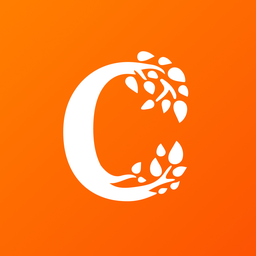
Full access? Get Clinical Tree
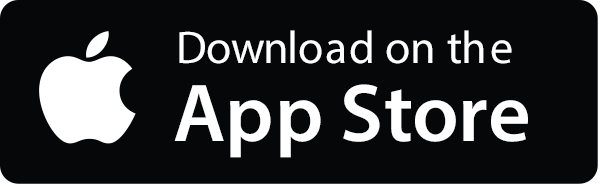
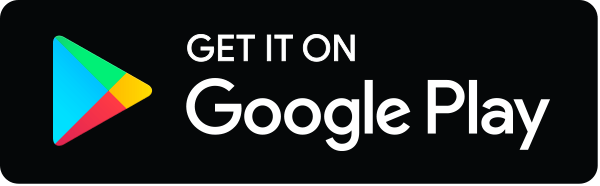