© Springer International Publishing Switzerland 2017
Cataldo Doria (ed.)Contemporary Liver TransplantationOrgan and Tissue Transplantation10.1007/978-3-319-07209-8_2525. Cell Therapy for Liver Failure: A New Horizon
(1)
Medistem Panama, Inc, Panama City, Panama
(2)
Riordan-McKenna Institute, Southlake, TX, USA
(3)
Aidan Foundation, Chandler, AZ, USA
Abstract
Liver failure is the seventh largest cause of death in industrialized countries. The only available cure, liver transplantation, is severely limited by a lack of donors and further complicated by the adverse effects of chronic immune suppression. Molecular mechanisms associated with the process of liver regeneration and the role of various progenitor cells in healing injury will be discussed in this chapter. Preclinical and clinical data will be reviewed from studies involving bone marrow mesenchymal stem cells (BM-MSC), adipose tissue MSC (AT-MSC), and bone marrow mononuclear cells (BMMC) including their purified subsets. From analyzing published clinical trials, it appears that there is some efficacy utilizing autologous cells, but these seem to be limited to a timeframe of less than a year. Promising sources of MSC such as the umbilical cord and fetal liver cells which have been used in allogeneic settings will also be described.
Keywords
Liver failureLiver regenerationStem cellMesenchymal stem cellsCell therapyFetal liver cellsProgenitor cellsIntroduction
Liver failure is a serious and, if untreated, fatal medical condition that occurs as a result of a number of acute and chronic clinical inciting factors, including drug-/alcohol-induced hepatotoxicity, viral infections, vascular injury, autoimmune disease, or genetic predisposition (Kelso 2008). Manifestations of liver failure include fulminant acute hepatitis, chronic hepatitis, or cirrhosis. Subsequent to various acute insults to the liver, the organ regenerates due to its unique ability to self-renew. If the insult is continuously occurring, the liver’s capacity to regenerate new cells is overwhelmed, and fibrotic nonfunctional tissue is deposited, diminishing the functional capacity of the hepatic parenchyma. The subsequent reduction of hepatocyte function can give rise to metabolic instability combined with disruption of essential bodily functions (i.e., energy supply, acid–base balance, and coagulation) (Bernuau et al. 1986; Farci et al. 1996; Navarro and Senior 2006). If not rapidly addressed, complications of hepatic dysfunction such as uncontrolled bleeding and sepsis occur, and dependent organs such as the brain and kidneys cease to function because of accumulation of toxic metabolites (Sargent 2006). In critical cases, such as when patients progress to acute-on-chronic liver failure (ACLF), liver transplant is considered to be the standard treatment. However, it is often extremely difficult to obtain a suitable donor, and many complications can arise after transplantation, including rejection and long-term adherence to immunosuppressant regimes (Kisseleva et al. 2010; Wu et al. 2008).
Liver Regeneration
Interest in regenerative approaches toward liver failure is not only due to the unmet medical need for novel interventions but is also based on the inherent ability of the liver to self-regenerate. It has been demonstrated that up to 70 % resection of the liver results in complete regeneration (Fausto et al. 2006; Michalopoulos 2011). Clinically, the potent regenerative ability of the liver allows for procedures such as living donor transplantation, two-stage hepatectomies, and split liver transplantation, which would be impossible with other organs that do not possess the potent inherent regenerative properties of the liver (Adam et al. 2000; Brown 2008; Clavien et al. 2007). However, liver regeneration is a tightly regulated process which adapts to the size of each specific body. In a partial hepatectomy, the liver will only regenerate to the original size, without hypertrophy. Transplantation medicine provides further insight into the tight regulation of liver regeneration. For example, smaller livers transplanted in proportionally larger recipients take on a larger size and vice versa (Michalopoulos 2013; Van Thiel et al. 1987).
Mechanistically, the process of liver regeneration occurs in three broad phases: (a) priming, (b) proliferation, and (c) termination (Fausto et al. 2006). It is important to note that hepatocytes are not terminally differentiated cells but cells that reside in a state of proliferative quiescence. Specifically, they share features with other regenerative cells such hematopoietic stem cells, in that they are normally in the G0 phase of cell cycle. This is altered during liver regeneration, which is described below.
During the priming phase, numerous injury signals are generated as a result of the underlying injury; these include activators of Toll-like receptors, complement degradation products, and damage-associated molecular patterns (DAMPs). These signals stimulate various cells, primarily Kupffer cells, to produce cytokines and growth factors such as interleukin-6 (IL-6), tumor necrosis factor-alpha (TNF-alpha), and hepatocyte growth factor (HGF), which induce entry of hepatocytes into cell cycle. The importance of these molecular signals in the initiation of liver regeneration is highlighted by knockout studies. Cressman et al. (1996) demonstrated blockade of liver regeneration in a partial hepatectomy IL-6 knockout model that was associated with blunted exit from the G0 phase of cell cycle in hepatocytes of these mice but not in non-parenchymal liver cells. Furthermore, they conclusively showed the importance of IL-6 in that a single preoperative dose of recombinant IL-6 restored post-injury hepatocyte entry into G1/2 to levels observed in wild-type mice and restored biochemical function (Cressman et al. 1996). Nuclear factor kappa-light-chain-enhancer of activated B cells (NF-kappa B) is a major downstream effector of various inflammatory cytokines including TNF-alpha and IL-6. Malato et al. (2008) generated hepatic-specific knockout mice in which the inhibitor of NF-kappa B, inhibitor of nuclear factor kappa B kinase subunit beta (IKK2), was ablated, thus giving rise to a higher level of background NF-kappa B activation. In these mice, partial hepatectomy resulted in accelerated entry of hepatocytes into cell cycle (Malato et al. 2008). The role of a variety of inflammatory or “danger”-associated pathways in the initial priming of hepatocyte proliferation after injury has been confirmed using DNA microarray analysis of genes associated with these signaling pathways such as STAT, p38MAPK, and Ras/ERK (Li et al. 2014).
The proliferation phase of hepatic regeneration is associated with “primed” hepatocytes leaving the G1 stage of cell cycle and entering the S phase, which is accompanied by phosphorylation of the retinoblastoma protein (pRb) and by upregulated expression of a number of proliferation-associated genes including cyclin E, cyclin A, and DNA polymerase (Fan et al. 1995; Spiewak Rinaudo and Thorgeirsson 1997). Key cytokines involved in stimulation of proliferation of the hepatocytes include HGF and epidermal growth factor (EGF). HGF is produced by mesenchymal cells, hepatic stellate cells, and liver sinusoidal endothelial cells as a proprotein, which acts both systemically and locally (DeLeve 2013; Maher 1993). Systemic elevations in HGF are observed after partial hepatectomy (Matsumoto et al. 2013), whereas local HGF is released from its latent form which is often bound to extracellular matrix proteins (Nakamura et al. 2011). Activation of HGF occurs typically via enzymatic cleavage mediated by urokinase-type plasminogen activator (uPA) (Mars et al. 1995; Shanmukhappa et al. 2006). The importance of HGF in the proliferation phase of liver regeneration is observed in animals where the HGF receptor c-MET is conditionally inactivated, displaying a reduction in hepatocyte entry into the S phase of cell cycle post-injury (Borowiak et al. 2004). EGF signaling has also been demonstrated to be involved in entry into the proliferative phase post-injury. Natarajan et al. (2007) performed perinatal deletion of epithelial growth factor receptor (EGFR) in hepatocytes prior to partial hepatectomy. They showed that, after hepatic injury, mice lacking EGFR in the liver had an increased mortality accompanied by increased levels of serum transaminases indicating liver damage. Liver regeneration was delayed in the mutants because of reduced hepatocyte proliferation. Analysis of cell cycle progression in EGFR-deficient livers indicated a defective G(1)-S phase entry with delayed transcriptional activation and reduced protein expression of cyclin D1, followed by reduced cyclin-dependent kinase 2 and cyclin-dependent kinase 1 (Natarajan et al. 2007).
The termination phase of liver regeneration occurs when the normal liver mass/body weight ratio of 2.5 % has been restored (Nygard et al. 2012). While in the priming phase of liver regeneration, several inflammatory cytokines are critical, in the termination phase, anti-inflammatory cytokines such as IL-10 (Mosser and Zhang 2008) are upregulated, which dampen proliferative stimuli (Yin et al. 2011). Additionally, cytokines with direct antiproliferative activity such as TGF-beta are generated, which results in cell cycle arrest of proliferating hepatocytes.
While classical liver regeneration is mediated by hepatocytes (Fausto et al. 2006; Miyaoka and Miyajima 2013) in certain situations, such as in liver failure, the ability of the hepatocytes to mediate regeneration is limited, and liver progenitor cells (LPCs) must carry out the process. The concept of a LPC taking over regenerative function when hepatocyte multiplication is stunted was first demonstrated in 1956 when Farber treated rats with various liver carcinogens that blocked division of hepatocytes (Farber 1956). He discovered the existence of “oval cells” which were subsequently demonstrated to act as LPCs having the ability to differentiate into both hepatocytes and biliary cells (Evarts et al. 1987). LPCs are found in the canals of Hering and bile ductules in human liver and found increased in patients with chronic liver disease (Libbrecht and Roskams 2002). It is unclear what the origin of LPCs is, whether they derive from local cells or directly from MSC (Banas et al. 2007), particularly bone marrow-derived MSC (Petersen et al. 1999), but the cellular mechanisms are poorly understood (Margini et al. 2014). In 2000, Theise et al. (2000b) found hepatocytes and cholangiocytes derived from extrahepatic circulating stem cells in the livers of female patients who had undergone therapeutic bone marrow transplantations. In the two female recipients from male donors and four male recipients from female donors, hepatocyte and cholangiocyte engraftment ranged from 4 % to 43 % and from 4 % to 38 %, respectively (Theise et al. 2000b).
Given the potent regenerative nature of the liver, combined with the possibility that extrahepatic cellular sources may contribute to regeneration, numerous attempts have been made to utilize cellular therapy for treatment of liver failure. The original hepatic cellular therapies involved the administration of allogeneic hepatocytes, which was initially attempted in animal models more than 30 years ago and is experimentally used clinically. Unfortunately, major hurdles exist that block this procedures from routine use, specifically (a) low number of suitable donors; (b) extremely poor hepatocyte viability after transplantation, with some groups as low as 30 %; and (c) need for continuous immune suppression which possesses inherent adverse effects (Filippi and Dhawan 2014).
Mesenchymal Stem Cells
Mesenchymal stem cells (MSC) are adult stem cells with self-renewing abilities (Jackson et al. 2007) and have been shown to differentiate into a wide range of tissues including mesoderm and nonmesoderm (Jackson et al. 2007; Pittenger et al. 1999), including hepatocytes (Banas et al. 2009; Cho et al. 2009; Hong et al. 2005; Ishikawa et al. 2010; Lee et al. 2004; Seo et al. 2005). MSC are capable of entering and maintaining satellite cell niches, particularly in hematopoiesis (Crisan et al. 2008; Tavian and Peault 2005), and are key in tissue repair and regeneration, aging, and regulating homeostasis (Aggarwal and Pittenger 2005; Caplan 2007; Chamberlain et al. 2007a; Peault et al. 2007). In the case of liver failure, MSCs can aid in regeneration of hepatic tissue (Banas et al. 2008; Chang et al. 2009; Kharaziha et al. 2009; Kuo et al. 2008; Lu et al. 2006; Mohamadnejad et al. 2007; Terai et al. 2006), and their interactions with the immune system (Chang et al. 2006; Iyer and Rojas 2008; Nauta and Fibbe 2007; Shi et al. 2011; Uccelli et al. 2007; Wolbank et al. 2007; Wolf and Wolf 2008) have potential as adjuvants during organ transplants (Sordi and Piemonti 2011), including liver transplantation (Popp et al. 2009).
MSC were discovered in 1970 by Friedenstein et al., who demonstrated that bone marrow (BM) contained both hematopoietic stem cells (HSCs), which are nonplastic adherent, and a population of a more rare adherent cell. The adherent cells were able to form single-cell colonies and were referred to as stromal cells. Those stromal cells, which are capable of self-renewal and expansion in culture, are now referred to as mesenchymal stem cells (MSC). Friedenstein was the first to show that MSC could differentiate into mesoderm and to demonstrate their importance in controlling the hematopoietic niche (Friedenstein et al. 1974).
In the 1980s, more research on MSC found that they could differentiate into muscle-, cartilage-, bone-, and adipose-derived cells (Caplan 1986). Caplan (1991) showed that MSC are responsible for bone and cartilage regeneration induced by local cuing and genetic potential.
In the 1990s, Pittenger et al. isolated MSC from bone marrow and found that they retained their multilineage potential after expanding into selectively differentiated adipocytic, chondrocytic, or osteocytic lineages (Pittenger et al. 1999). Likewise, Kopen et al. showed that bone marrow MSC differentiated into neural cells when exposed to the brain microenvironment (Kopen et al. 1999). In 1999, Petersen et al. found that bone marrow-derived stem cells could be a source of hepatic oval cells in a rat model (Petersen et al. 1999). Specifically, they used male-to-female bone marrow transplant and subsequently induced blockade of hepatocyte proliferation by administration of a hepatotoxin followed by partial hepatectomy. As previously described, this procedure stimulates proliferation of LPC or “reserve cells” which generate new hepatocytes, such cells having been previously identified as oval cells. Subsequent to the hepatectomy, Y chromosome, dipeptidyl peptidase IV enzyme, and L21-6 antigen were used to identify the newly generated oval cells and their hepatocytic progeny to be of bone marrow origin.
The first decade of the twenty-first century saw a surge of research on MSC, leading to a greater understanding of their nature and of the cellular process behind regeneration (Caplan 2007; Chamberlain et al. 2007a; Jackson et al. 2007). In 2005, Teratani et al. identified growth factors allowing hepatic fate specification in mice and showed that embryonic stem cells could differentiate into functional hepatocytes (Teratani et al. 2005). In 2007, Chamberlain et al. generated human hepatocytes from clonal MSC in fetal sheep hepatic tissue, differentiating into hepatocytes both throughout the liver parenchyma and the periportal space (Chamberlain et al. 2007b). The lack of need for donor matching compounded by their ease of expansion and the standardized protocols for manufacturing and administration make MSCs attractive for clinical development. Of particular interest for liver conditions is the observation that intravenous administration of MSC results in a primary homing of cells to the lung, followed by homing and retention to the liver (Gao et al. 2001). A unique property of MSC is their apparent hypoimmunogenicity and immune modulatory activity (Le Blanc and Ringden 2007), which is present in MSC derived from various sources (Keyser et al. 2007). This is believed to account for the ability to achieve therapeutic effects in an allogeneic manner. Allogeneic bone marrow-derived MSC have been used by academic investigators with clinical benefit in the treatment of diseases such as graft-versus-host disease (GVHD) (Ball et al. 2008; Le Blanc et al. 2004, 2008; Muller et al. 2008; Ning et al. 2008; Ringden et al. 2006), osteogenesis imperfecta (Horwitz et al. 2002), Hurler syndrome, metachromatic leukodystrophy (Koc et al. 2002), and acceleration of hematopoietic stem cell engraftment (Ball et al. 2007; Lazarus et al. 2005; Le Blanc et al. 2007). The company Athersys has successfully completed Phase I safety studies using allogeneic bone marrow MSC and is now in efficacy seeking clinical trials (Phase II and Phase III) for multiple sclerosis, Crohn’s disease, and graft-versus-host disease using allogeneic bone marrow-derived MSC. Intravenous administration of allogeneic MSC by Osiris was also reported to induce a statistically significant improvement in cardiac function in a double-blind study (Osiris Therapeutics 2007).
Currently, there are several MSC-based therapies that have received governmental approvals including ProchymalTM, registered in Canada and New Zealand for treatment of graft-versus-host disease (Kellathur and Lou 2012; Kurtzberg et al. 2014). Although in terms of clinical translation bone marrow MSC are the most advanced, several other sources of MSC are known which possess various properties that may be useful for specific conditions. Bone marrow is also a source for hematopoietic stem cells (HSCs), which have also been used for liver regeneration. Likewise, human placenta is an easily accessible source of abundant MSC, which can be differentiated in vitro. Finally, MSC with tissue regenerative abilities can also be isolated from adipose tissue and induced to hepatocytes in large numbers.
Bone Marrow MSC for Treatment of Liver Failure
Early studies have suggested that out of the hepatic regenerative cells found in bone marrow, the MSC component is the most regenerative cell type as compared to other cell types such as hematopoietic stem cells (Cho et al. 2009). Given that BM-MSC are capable of differentiating into various tissues in vitro, combined with the putative bone marrow origin of the hepatic-repairing oval cell (Petersen et al. 1999), investigators sought to determine whether BM-MSC could be induced to differentiate into hepatocyte cells in vitro through culture in conditions that would imitate hepatic regeneration. Lee et al. (2004) developed a 2-step protocol for hepatocyte differentiation using culture in hepatocyte growth factor, followed by oncostatin M. After 4 weeks of induction, the investigators reported the spindle-like BM-MSC taking a cuboidal morphology, which is characteristic of hepatocytes. Furthermore, the differentiating cells were seen to initiate expression of hepatic-specific genes in a time-dependent manner correlating with morphological changes. From a functional perspective, the generated hepatocytes exhibited features of liver cells, specifically albumin production, glycogen storage, urea secretion, uptake of low-density lipoprotein, and phenobarbital-inducible cytochrome P450 activity (Lee et al. 2004). To improve yield and potency of BM-MSC-generated hepatocytes, Chen et al. utilized conditioned media from cultured hepatocytes as part of the differentiation culture conditions. They reported that BM-MSC cultures in the differentiation conditions started taking an epithelioid, binucleated morphology at days 10 and 20. Gene assessment revealed increase in AFP, HNF-3beta, CK19, CK18, ALB, TAT, and G-6-Pase mRNA, which was confirmed at the protein levels. Additionally, the cells started taking a functional phenotype similar to hepatocytes. The hepatocyte-like cells by culture in conditioned medium further demonstrated in vitro functions characteristic of liver cells, including glycogen storage and urea secretion activities. Upon transplantation to immune-deficient animals exposed to chemically induced liver injury (Chen et al. 2007), restoration of albumin activity and suppression of liver enzymes were seen, demonstrating in vivo relevance of these artificially generated hepatic-like cells. In accordance with the concept that injured tissue mediates MSC activation and subsequent repair, Mohsin et al. (2011) demonstrated that coculture of BM-MSC with chemically injured hepatocytes augments hepatic differentiation as compared to coculture with naïve hepatocytes.
Based on in vitro differentiation, as well as the possibility of MSC producing cytokines such as HGF (Lange et al. 2005; Li et al. 2012; Soleymaninejadian et al. 2012), which are known to stimulate hepatic regeneration and/or decrease hepatocyte apoptosis (Enriquez-Cortina et al. 2013; Francois et al. 2013; Hua et al. 2012; Kaldenbach et al. 2012; Kroy et al. 2014; Lee et al. 2010b, 2012; Li et al. 2013), several animal studies were conducted using BM-MSC in models of liver injury. Fang et al. (2004) utilized a carbon tetrachloride-induced hepatic injury model to assess the effects of systemically administered BM-MSC on fibrosis and hepatocyte demise. It was found that MSC infusion after exposure to the hepatotoxin significantly reduced liver damage and collagen deposition. Levels of hepatic hydroxyproline and serum fibrosis markers in mice receiving cells were significantly lower compared with those of control mice, supporting the possibility of a concurrent protective and regenerative effect. Histologic examination suggested that hepatic damage recovery was accelerated in the treated mice. Donor cell engraftment and possible in vivo hepatic differentiation were supported by immunofluorescence, polymerase chain reaction, and fluorescence in situ hybridization analysis, which demonstrated donor-derived cells possessing epithelium-like morphology and expressed albumin (Fang et al. 2004). Interestingly, the amount of engrafted cells was minute and could not explain the functional recovery of serum albumin, suggesting the possibility of paracrine effects. A subsequent study using the same carbon tetrachloride model demonstrated that BM-MSC administration resulted in reactive oxygen species ex vivo, reduced oxidative stress in recipient mice, and accelerated repopulation of hepatocytes after liver damage (Kuo et al. 2008). To optimize the route of administration, Zhao et al. (2012) assessed intravenous, intrahepatic, and intraperitoneal administration of BM-MSC in rats treated with carbon tetrachloride. Functional recovery was most profound in the intravenous administration group, which was correlated with increased IL-10 and decreased IL-1, TNF-alpha, and TGF-beta. Furthermore, in vivo differentiation of the BM-MSC was observed based on expression of α-fetoprotein, albumin, and cytokeratin 18 in cells derived from donor origin (Zhao et al. 2012).
In order to assess whether the therapeutic effects of BM-MSC are specific to the carbon tetrachloride model or whether they may be extrapolated to other models of hepatic injury, investigators assessed the usefulness of BM-MSC in hepatectomy recovery models. While recovery is generally observed after two-thirds or 70 % hepatectomy, 90 % hepatectomy is lethal in rats. In one study, BM-MSC were differentiated in vitro by culture on Matrigel with hepatocyte growth factor and fibroblast growth factor-4 into cells expressing hepatocyte-like properties. Specifically, the cells expressed a hepatic-like cuboidal morphology and were positive for albumin, cytochrome P450 (CYP)1A1, CYP1A2, glucose 6-phosphatase, tryptophane-2,3-dioxygenase, tyrosine aminotransferase, hepatocyte nuclear factor (HNF) 1 alpha, and HNF4alpha. Intrasplenic administration of differentiated cells subsequent to the 90 % hepatectomy resulted in the prevention of lethality (Miyazaki et al. 2007). Another study confirmed the efficacy of BM-MSC at accelerating post-hepatectomy liver regeneration subsequent to intraportal administration. Regenerative effects were associated with upregulation of HGF expression in the newly synthesized tissue (Kaibori et al. 2014). It is interesting that the regenerative effects of BM-MSC are observed not only in acute settings but also in chronic conditions leading to liver failure. Nonalcoholic steatohepatitis (NASH) is a precursor to cirrhosis and is characterized by lipid accumulation, hepatocyte damage, leukocyte infiltration, and fibrosis. It was demonstrated that in C57BL/6 mice chronically fed with high-fat diet, the intravenous administration of BM-MSC resulted in reduction of plasma levels of hepatic enzyme, hepatomegaly, liver fibrosis, inflammatory cell infiltration, and inflammatory cytokine gene expression, as compared to control mice (Ezquer et al. 2011). Overall, these data suggest that BM-MSC have some reparative and/or regenerative activity on livers that are damaged in either chronic or acute settings.
Additional animal studies have been conducted in both chronic and acute liver toxicity settings. For example, Hwang et al. (2012) treated Sprague–Dawley rats with 0.04 % thioacetamide (TAA)-containing water for 8 weeks, and BM-MSC were injected into the spleen with the intent of transsplenic migration into the liver. Ingestion of TAA for 8 weeks induced micronodular liver cirrhosis in 93 % of rats. Examination of MSC microscopically revealed that the injected cells were diffusely engrafted in the liver parenchyma, differentiated into CK19 (cytokeratin 19)- and Thy1-positive oval cells and later into albumin-producing hepatocyte-like cells. MSC engraftment rate per slice was measured as 1.0–1.6 %. MSC injection resulted in apoptosis of hepatic stellate cells and resultant resolution of fibrosis but did not cause apoptosis of hepatocytes. Given that stellate cells are responsible for matrix deposition and fibrosis (Novo et al. 2014; Xu et al. 2014), this is an interesting observation. Injection of MSC treated with HGF in vitro for 2 weeks, which became CD90 negative and CK18 positive, resulted in chronological advancement of hepatogenic cellular differentiation by 2 weeks and decrease in anti-fibrotic activity. Mechanistically, it appeared that the BM-MSC directly differentiated to oval cells and hepatocytes, which was associated with repair of damaged hepatocytes, intracellular glycogen restoration, and resolution of fibrosis.
An acute model of liver failure is produced by administration to animals of d-galactosamine, a TNF-alpha-stimulating hepatotoxin (Wu et al. 2014), and lipopolysaccharide (LPS), a potent inflammatory stimulus that replicates translocation of gut bacteria often seen in liver failure (Lindros and Jarvelainen 2005; Sandler et al. 2011). In this model, it was demonstrated that administration of BM-MSC in pretreated rats resulted in reduction of ALT, AST, caspase-1 and IL-18 proteins, and mRNA as compared to the control group (Yuan et al. 2013). Mechanistic elucidation at a cellular level demonstrated that the injected BM-MSC were inhibiting hepatocyte apoptosis. Interestingly, the authors also found that recovering animals possessed higher levels of VEGF protein as compared to non-treated animals. This is intuitively logical given that VEGF is a key cytokine in the angiogenesis cascade, and angiogenesis seems to be required in the regression of liver failure (Kajdaniuk et al. 2011; Sturm et al. 2004; Tekkesin et al. 2011; Ueno et al. 2006). Using the same d-galactosamine/LPS model, Sun et al. (2014) sought to identify optimal route of delivery for BM-MSC. They divided the rats into the following groups: (a) hepatic artery injection group, (b) portal vein injection group, (c) tail vein injection group, and (d) intraperitoneal injection group. They found that compared with the control group, ALT, AST, and damage to the liver tissue improved in vivo in the hepatic artery group, the portal vein group, and the tail vein group. The expression of PCNA and HGF in the liver was higher, and caspase-3 expression was lower in the hepatic artery injection group, the portal vein injection group, and the tail vein injection group than in the intraperitoneal injection and control groups. The BrdU-labeled BM-MSC were only observed homing to the liver tissue in these three groups. However, no significant differences were observed between these three groups. Liver function was improved following BM-MSC transplantation via three endovascular implantation methods (through the hepatic artery, portal vein, and vena caudalis). These data suggest that intrahepatic artery injection was most effective and that intraperitoneal administration is ineffective. A large animal study using similar hepatotoxins was performed in the pig. Li et al. (2014) administered 3 × 10(7) human BM-MSC via the intraportal route or peripheral vein immediately after d-galactosamine injection, and a sham group underwent intraportal transplantation (IPT) without cells (IPT, peripheral vein transplantation [PVT], and control groups, respectively, n = 15 per group). All of the animals in the PVT and control groups died of FHF within 96 h. In contrast, 13 of 15 animals in the IPT group achieved long-term survival (>6 months). Immunohistochemistry demonstrated that transplanted human BM-MSC-derived hepatocytes in surviving animals were widely distributed in the hepatic lobules and the liver parenchyma from weeks 2–10. Thirty percent of the hepatocytes were BM-MSC derived. However, the number of transplanted cells decreased significantly at week 15. Only a few single cells were scattered in the regenerated liver lobules at week 20, and the liver tissues exhibited a nearly normal structure. These data suggest that intraportal delivery may be ideal and also reinforce the notion that MSC may be transplanted across allo- and xeno-barriers without need for immune suppression.
Clinical trials utilizing BM-MSC have shown an excellent safety profile, with various levels of efficacy in liver failure. Mohamadnejad et al. (2007) conducted a 4-patient study with decompensated liver cirrhosis. Patient bone marrow was aspirated, mesenchymal stem cells were cultured, and a mean 31.73 × 10(6) mesenchymal stem cells were infused through a peripheral vein. There were no side effects in the patients during follow-up. The model for end-stage liver disease (MELD) scores of patients 1 and 4 improved by four and three points, respectively, by the end of follow-up. Furthermore, the quality of life of all four patients improved by the end of follow-up. Using the SF-36 questionnaire, the mean physical component scale increased from 31.44 to 65.19, and the mean mental component scale increased from 36.32 to 65.55. Another study treated 8 patients (four hepatitis B, one hepatitis C, one alcoholic, and two cryptogenic) with end-stage liver disease having MELD score greater than or equal to 10 were included. Autologous bone marrow was taken from the iliac crest. Approximately 30–50 million ex vivo expanded BM-MSC were injected into the peripheral or the portal vein. Subsequent to experiment, the MELD score was decreased from 17.9+/−5.6 to 10.7+/−6.3 (P < 0.05) and prothrombin complex from international normalized ratio 1.9+/−0.4 to 1.4+/−0.5 (P < 0.05). Serum creatinine decreased from 114+/−35 to 80+/−18 μmol/l (P < 0.05). This trial supports the safety with signal of efficacy of the BM-MSC activity in liver failure clinically.
A larger trial of autologous BM-MSC focused on patients with liver failure associated with hepatitis B infection (Peng et al. 2011). Part of the rationale was previous studies showing that BM-MSC-derived hepatocytes are resistant to hepatitis B infection (Xie et al. 2009). Peng et al. (2011) treated 53 patients and as controls used 105 patients matched for age, sex, and biochemical indexes, including alanine aminotransferase (ALT), albumin, total bilirubin (TBIL), prothrombin time (PT), and MELD score. In the 2–3-week period after cell administration, efficacy was observed based on the levels of ALB, TBIL, and PT and MELD score, compared with those in the control group. Safety of the procedure was demonstrated in that there were no differences in incidence of hepatocellular carcinoma (HCC) or mortality between the treated and control groups at 192 weeks. Unfortunately, liver function between the two groups was also similar at 192 weeks, suggesting the beneficial effects of BM-MSC were transient in nature. Supporting the possibility of transient effects of BM-MSC was a 27-patient study in patients with decompensated cirrhosis in which 15 patients received BM-MSC and 12 patients received placebo. The absolute changes in Child scores, MELD scores, serum albumin, INR, serum transaminases, and liver volumes did not differ significantly between the MSC and placebo groups at 12 months of follow-up. Unfortunately, the publication did not provide 3- or 6-month values (Mohamadnejad et al. 2013). In contrast, a more recent study administered BM-MSC into 12 patients (11 males, 1 female) with baseline biopsy-proven alcoholic cirrhosis who had been alcohol free for at least 6 months (Jang et al. 2014). A 3-month assessment of histological improvement and reduction of fibrosis was quantified according to the Laennec fibrosis scoring scale in 6 of 11 patients. Additionally, at 3 months post-cell administration, the Child–Pugh score improved significantly in ten patients, and the levels of transforming growth factor-β1, type 1 collagen, and α-smooth muscle actin significantly decreased (as assessed by real-time reverse transcriptase polymerase chain reaction) after BM-MSC therapy. Overall, the different underlying conditions, route of administration, and time points of assessments between studies make it difficult to draw solid conclusions; it appears that some therapeutic effect exists, although longevity of effect is not known.
One possibility for the lack of long term efficacy in the previous study may be inappropriate level of hepatocyte differentiation in vivo, Amer et al. (2011) conducted a clinical trial where BM-MSC were pre-differentiated toward the hepatocyte lineage by a culture cocktail containing HGF. They conducted a 40-patient trial in hepatitis C patients in which 20 patients were treated with partially differentiated cells either intrasplenically or intrahepatically and 20 patients received placebo control. At the 3- and 6-month time points, a significant improvement in ascites, lower limb edema, and serum albumin, over the control group, was observed. Additionally, significant benefit was quantified in the Child–Pugh and MELD scores. No difference was observed between intrahepatic and intrasplenic administration. This study demonstrates the potential of semi-differentiated hepatocytes from BM-MSC to yield therapeutic benefit without reported adverse effects.
Out of the BM-MSC studies described, one potential reason for relatively mediocre results could be the fact that autologous cells were utilized in all of the studies. While autologous MSC possess the benefit of lack of immunogenicity, a drawback may be a relative dysfunction of these cells given the poor health condition of the patients. Several studies have demonstrated that MSC from patients suffering from chronic conditions possess inhibited regenerative activity when compared with MSC from healthy donors (Bozdag-Turan et al. 2012; Cipriani et al. 2007; Rodriguez-Menocal et al. 2012; Sugihara et al. 2007; Teraa et al. 2013; Walter et al. 2005; Zhuo et al. 2010).
Adipose-Derived MSC for Treatment of Liver Failure
Adipose tissue is an attractive alternative to bone marrow as a source of stem cells for treatment of degenerative conditions in general and liver failure specifically (Ishikawa et al. 2010; Puglisi et al. 2011) for the following reasons: (a) extraction of adipose-derived cells is a simpler procedure that is much less invasive than bone marrow extraction; (b) adipose tissue contains a higher content of mesenchymal stem cells (MSC) as compared to bone marrow; therefore, shorter in vitro expansion times are needed; and (c) MSC from adipose tissue do not decrease in number with aging (Mosna et al. 2010; Strioga et al. 2012; Yi and Song 2012). Adipose derived MSC were originally described by Zuk et al. who demonstrated that the stromal vascular fraction (SVF) of adipose tissue contains large numbers of cells that could be induced to differentiate into adipogenic, chondrogenic, myogenic, and osteogenic lineages and morphologically resembled MSC (Zuk et al. 2001). Subsequent to the initial description, the same group reported that in vitro expanded SVF-derived cells had surface marker expression similar to bone marrow-derived MSC, displaying expression of CD29, CD44, CD71, CD90, CD105/SH2, and SH3 and lacking CD31, CD34, and CD45 expression (Zuk et al. 2002). This suggested that SVF-expanded adherent cells were indeed members of the MSC family, a notion that has subsequently gained acceptance (Bassi et al. 2012; Ong and Sugii 2013; Tallone et al. 2011). To date, clinical trials on adipose-derived cells have all utilized ex vivo expanded cells, which share properties with bone marrow-derived MSC (Fang et al. 2006, 2007a, b; Garcia-Olmo et al. 2005, 2008; Stillaert et al. 2008). Preparations of MSC expanded from adipose tissue are equivalent or superior to bone marrow in terms of differentiation ability (Hayashi et al. 2008; Noel et al. 2008), angiogenesis-stimulating potential (Kim et al. 2007), and immune modulatory effects (Keyser et al. 2007; Lin et al. 2012).
In the area of liver failure, Banas et al. (2009) created a 13-day in vitro differentiation protocol to generate hepatocyte-like cells from human adipose tissue MSC (AT-MSC). The differentiated cells possessed a hepatocyte-like morphology and phenotypically resembled primary hepatocytes. Administration of the cells in a carbon tetrachloride-induced liver failure model resulted in diminished liver injury, AST, ALT, as well as ammonia. Unfortunately, comparison with BM-MSC was not performed. A subsequent study utilized AT-MSC that were not differentiated and injected into the tail vein (Yukawa et al. 2009). Administration of cells led to death in 4 of 6 mice due to lung infarction, presumably as a result of cell accumulation in pulmonary microcapillaries. To overcome this, the investigators utilized a combination of AT-MSC and heparin. This resulted in a trend that did not reach significance for reduced ALT, AST, and LDH in the treated group. It was demonstrated in a subsequent tracking study by the same group that heparin decreased pulmonary retention and increased hepatic retention by 30 % (Yukawa et al. 2012). In order to elucidate whether alternative routes of AT-MSC administration may augment therapeutic activities, Kim et al. (2011) assessed intravenous, intrahepatic parenchyma, and intraportal vein delivery of cells in the same carbon tetrachloride model as utilized by the previous two experiments. They found that all three routes led to significant decrease in histological injury as well as AST, ALT, and ammonia. The most profound protective effects were observed in the intravenous group. One possible reason for statistical significant efficacy in this study and not in the previous study may be that in this study AT-MSC were injected at days 1 and 3 after carbon tetrachloride administration whereas the previous study involved only one injection. The previous AT-MSC experiments utilized human cells administered in animals; Deng et al. (2014) utilized syngeneic AT-MSC that were derived from mice transgenic with enhanced green fluorescent protein (eGFP) in mice treated with carbon tetrachloride. The survival rate of the cell-treated group significantly increased compared to the PBS group. Furthermore, the transplanted cells were well integrated into injured livers and produced albumin and cytokeratin-18. Overall, it appears that in the carbon tetrachloride model, both xenogeneic and syngeneic AT-MSC have therapeutic effects. However, standardization of protocols and models is needed to obtain a clearer picture of potency of effects.
Other models of hepatic injury have been utilized with AT-MSC. Salomone et al. (2013) assessed human AT-MSC transfected with eGFP in rats treated with a hepatotoxic dose of acetaminophen. It was found that AT-MSC infusion decreased AST, ALT, and prothrombin time to the levels observed in control rats. Furthermore, clinical signs of liver failure such as encephalopathy were not observed in treated animals. Histologically, control animals displayed lobular necrosis and diffuse vacuolar degeneration, which was not seen in the treated group. Mechanistically, transplanted AT-MSC induced an increase in antioxidant status and decrease in inflammatory cytokines in the recipients. Additionally, proliferation of endogenous hepatocytes was observed. These data suggest that AT-MSC effects on liver injury are not limited to carbon tetrachloride but may be more widespread. Indeed, another study utilized two chemicals that block hepatocyte regeneration together with partial hepatectomy. Specifically, using a model of a toxic liver damage in Sprague–Dawley rats, generated by repetitive intraperitoneal application of retrorsine and allyl alcohol followed by two-thirds partial hepatectomy, investigators assessed the regenerative effects of human AT-MSC. Six and twelve weeks after hepatectomy, animals were sacrificed and histological sections were analyzed. AT-MSC-treated animals exhibited significantly raised albumin, total protein, glutamic oxaloacetic transaminase, and LDH. The infused cells were found in histological sections up to 12 weeks after surgery. Although clinical studies of AT-MSC in liver disease have not been reported at the time of writing, one clinical trial (NCT01062750) is reported to be enrolling. This trial, run by Shuichi Kaneko of Kanazawa University in Japan, comprises of intrahepatic administration of AT-MSC.
Bone Marrow Mononuclear Cells and Isolated Subsets
Although numerous studies have examined the ability of MSC to induce hepatic regeneration, the original studies that demonstrated BM liver regenerative effects suggested that other cells in the BM compartment besides MSC may have therapeutic activities (Avital et al. 2002). Given that bone marrow mononuclear cells (BMMC) have demonstrated therapeutic activities in numerous ischemic and chronic conditions (de Vasconcelos Dos Santos et al. 2010; Fernandez-Aviles et al. 2004; Iihoshi et al. 2004; Nizankowski et al. 2005; Strauer et al. 2002; Tateishi-Yuyama et al. 2002), investigators sought to assess whether this mixture of cells would possess activity in animal models of liver failure. Terai et al. (2003) administered BMMC isolated from mice transgenic for GFP to mice whose livers where injured by carbon tetrachloride. It was observed that the transplanted GFP-positive BMMC migrated into the periportal area of liver lobules after 1 day and repopulated as much as 25 % of the recipient liver by 4 weeks. Interestingly, when mice were administered with BMMC but not carbon tetrachloride, no donor cells could be detected at 4 weeks, indicating that injury must be present for long-term hepatic retention. It appeared that the transplanted BMMC differentiated into functional mature hepatocytes, which would overtake function of hepatocytes from carbon tetrachloride-injured mice (Terai et al. 2003). A subsequent study by the same group examined the mechanisms of the anti-fibrotic and/or regenerative effect of the BMMC and found matrix metalloproteinase (MMP) activation to be involved (Sakaida et al. 2004). MMPs are important in liver regeneration not only because of their ability to cleave through fibrotic tissue in order to alter the local environment but also because of their role in angiogenesis, which is important for liver regeneration (Bellayr et al. 2009; Kawai et al. 2012; Malemud 2006). Interestingly, regression of liver fibrosis by dendritic cells also is mediated through MMP activation (Jiao et al. 2012).
One of the first clinical uses of BMMC in the liver involved purification of CD133-positive cells prior to administration, with the notion that CD133 selects for cells with enhanced regenerative potential (Handgretinger and Kuci 2013). Additionally, the CD133 subset of bone marrow cells may represent a hepatogenic precursor cell since cells of this phenotype are mobilized from the bone marrow subsequent to partial hepatectomy (Gehling et al. 2005; Harb et al. 2009; Zocco et al. 2011). Another interesting point is that CD133 has been reported by some to be expressed on oval cells in the liver, although the bone marrow origin is controversial (Rountree et al. 2007, 2011; Yovchev et al. 2007). In 2005, Esch et al. described 3 patients subjected to intraportal administration of autologous CD133(+) BMMC subsequent to portal venous embolization of right liver segments, used to expand left lateral hepatic segments. Computerized tomography scan volumetry revealed 2.5-fold increased mean proliferation rates of left lateral segments compared with a group of three consecutive patients treated without application of BMMC (am Esch et al. 2005). In 2012, the same group reported on 11 patients treated with this procedure and 11 controls. They found that mean hepatic growth of segments II/III 14 days after portal vein embolization in the group that received CD133 cells was significantly higher (138.66 mL ± 66.29) when compared with the control group (62.95 mL ± 40.03; P = 0.004) (am Esch et al. 2012). Post hoc analysis revealed a better survival for the group that received cells as compared to the control. A similar study by another group involved 6 patients receiving CD133 cells to accelerate left lateral segment regeneration, with 7 matched control patients. The increase of the mean absolute future liver remnant volume (FLRV) in the treated group (from 239.3 mL +/− 103.5 to 417.1 mL +/− 150.4) was significantly higher than that in the control group (from 286.3 mL +/− 77.1 to 395.9 mL +/− 94.1). The daily hepatic growth rate in the treated group (9.5 mL/d +/− 4.3) was significantly higher than in the control group (4.1 mL/d +/− 1.9) (P = 03). Furthermore, time to surgery was 27 days +/− 11 in the treated group and 45 days +/− 21 in the control group (P = 057) (Furst et al. 2007). These data suggest that in the clinical situation, CD133 cells isolated from BMMC appear to accelerate liver regeneration.
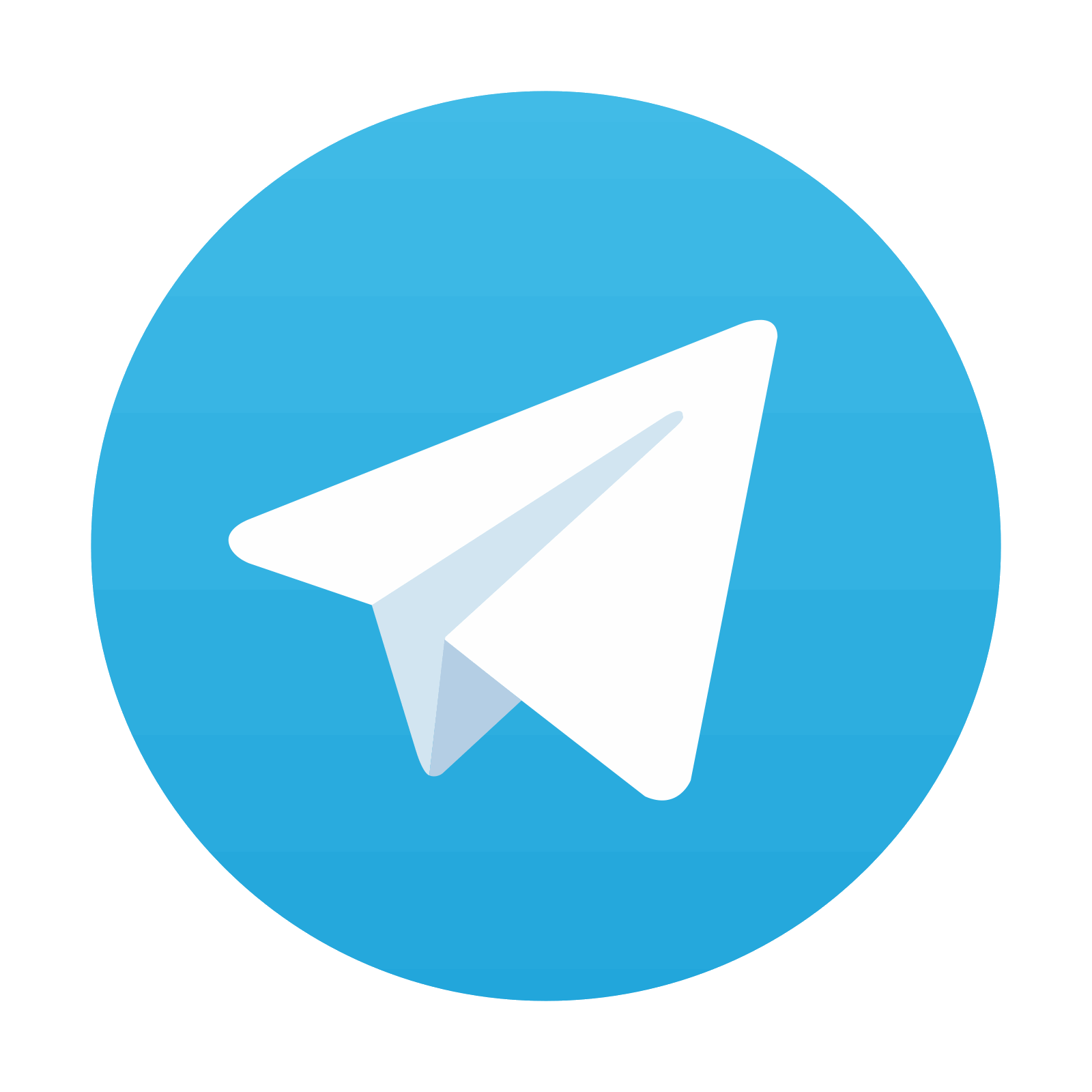
Stay updated, free articles. Join our Telegram channel
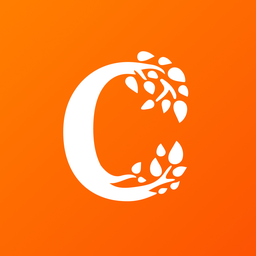
Full access? Get Clinical Tree
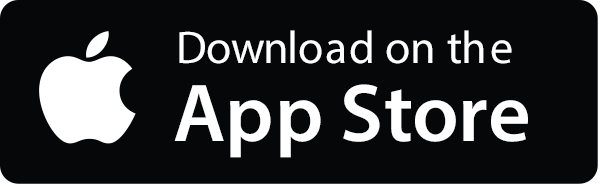
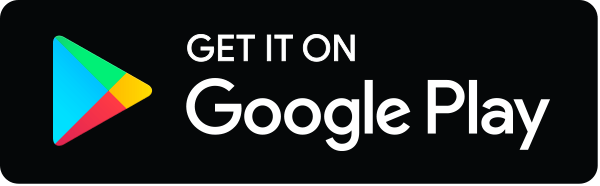