Outline
Introduction 245
Design Criteria for Creating a Bioartificial Niche 245
Chemistry of Bioartificial Niches 246
Fabrication Techniques 246
Chemical Cross-linking 248
Photo-cross-linking 248
Sol-gel Synthesis 248
Cryogelation 248
Spinning 248
Bioprinting 249
Centrifugal Casting 249
Microfabrication 250
Common Hydrogel Materials 250
Examples of Hydrogel Applications 251
Recruitment and Delivery of Stem and Progenitor Cells to and from Artificial Niches 252
Extracellular Scaffold as a Mold for Kidney Regeneration 252
Hydrogel Encapsulation of Endothelial Progenitor Cells Protects Cells from Cytotoxins and Improves Their Viability 253
Ongoing attempts to emulate the properties of stem cell niches using synthetic materials are summarized. An overarching theme of these studies is encapsulated in the idea that three-dimensional extracellular matrix or artificial scaffolds could meet at least some of niche requirements. The chemical composition of different scaffolds, their fabrication and physicochemical properties are described. In addition, some uses of such matrices for storage and delivery of different stem cells and the results of these preclinical studies are discussed.
Introduction
With the rapidly increasing demand for stem cells, the problems related to their storage and preservation, maintenance and expansion become ever more acute. The requirements for stem cell banking would place the need for preserving quiescence and stemness at the top of the list, whereas the requirements for transplantation of stem and progenitor cells would give preference to the possibility of increasing the mass of stem cells without losing their properties. Inevitably, the constellation of different requirements should orbit around the physiological ways of preserving stem cells, i.e. stem cell niches. The niches are defined as the umbrella microenvironment supporting cell attachment and quiescence by sheltering cells from proliferation and differentiation signals, enhancing cell survival, regulating stem cell division and renewal, and coordinating the population of resident stem cells to meet the actual requirements of an organ . The way in which these diverse functions are accomplished by the niches may vary from one type of stem cells and their niche to another, yet the general outline is preserved. It includes specific components of the extracellular matrix (ECM) that create a scaffold and a boundary to the niche, cell–cell and cell–matrix adhesion molecules, locally stored growth and/or quiescence factors, and a set of instructions for proliferation and/or migration that can be triggered on demand. The vicinity of vascular beds, often covered with sinusoidal endothelial cells, provides a portal for entry into the circulation.
Design Criteria for Creating a Bioartificial Niche
The design criteria for matrices for encapsulation of cells for cell therapy include not only technological criteria from chemistry, biology and engineering, but also real-world marketing, regulatory and financial constraints . A biocompatible material suitable for a three-dimensional (3D) bioartificial niche should be easy to use, have user-determined composition and compliance, and permit seamless transitions from in vitro to preclinical to clinical use. Replacing the complex native ECM environment with a minimalist semi-synthetic ECM allows cells to remodel the provisional matrix into a tissue-specific architecture . These semi-synthetic extracellular matrices (sECMs) are “living” biopolymers based on chemically modified hyaluronan (HA), a ubiquitous ECM glycosaminoglycan that is evolutionarily conserved from the simplest prokaryotes to complex tissues of eukaryotes. The sECMs allow inclusion of the appropriate biological cues needed to simulate the complexity of the ECM of a given tissue and offer a manufacturable, highly reproducible, flexible, FDA-approvable and affordable vehicle for cell expansion and differentiation in 3D.
For a biomaterial to create a bioartificial niche, it must recapitulate the principal functions of the natural ECM in orchestrating cell proliferation, migration, differentiation, angiogenesis and invasion . A bioartificial niche should orchestrate the integration of biochemical and mechanical cues in the cellular microenvironment and allow versatility in engineering a regenerative microenvironment . Specifically, the performance criteria should include: (i) experimental control of composition and compliance; (ii) control of bioresorption in vitro and in vivo; (iii) flexibility of physical form to allow for both cell encapsulation and biofabrication; (iv) batch-to-batch consistency; (v) ease of use at physiological temperature and pH; (vi) transparency for ease of visualization; and (vii) the ability to translate preclinical results into a clinical product. In addition, the pharmaceutical industry requires a bioartificial niche for drug evaluation purposes that is compatible with high-throughput screening (HTS) platforms . One solution is a modular platform of sECMs that enables the user to add soluble factors, attachment peptides, matricellular proteins, cross-linkers and macromomers in the combinations required for a given cell type and microenvironment .
Chemistry of Bioartificial Niches
The cornerstone idea behind artificial stem cell niches investigated thus far is in the generation of scaffolds with the properties of the ECM . In this regard, chemically engineered synthetic hydrogels (as opposed to natural substances like fibrin or collagen) have been extensively studied based on their mechanical and structural properties that emulate the ECM. The governing design principles include controlled degradation, local delivery of macromolecular regulators (growth factors, cytokines and adhesion molecules), and tolerance and integration into the native neighboring tissues.
A growing number of fibrous scaffolds and hydrogels is in development for the expansion of primary cells and progenitor cells in 3D . A hydrogel is a cross-linked network (of either natural or synthetic polymers) that is hydrophilic and swells substantially in water. Hydrogels can be as much as 95% water by volume when fully swelled . Hydrogels suffer from a lack of mechanical strength owing to the large amount of swelling observed in aqueous environments. Often, hydrogels are formed from a combination of different polymer chains where one serves to provide mechanical support for the gel, while the other adds favorable properties such as binding sites for cells or encapsulated biomolecules (such as growth factors or other proteins) . Hydrogels are sometimes formed as interpenetrating networks where two different polymers are separately cross-linked in the same reaction vessel .
Among the naturally derived biomaterials is an in situ cross-linkable sECM that is based on chemically modified HA . HA plays a critical role in morphogenesis , and chemically modified HA derivatives have generated considerable interest as biomaterials for tissue engineering . As specified in the design criteria above, this versatile sECM offers user-controllable composition and compliance, to which a variety of growth factors or matricellular proteins may be added . The elastic moduli of hydrogels can be tailored to match the cell type to achieve optimal regeneration of new tissue .
Among synthetic hydrogels, poly(ethylene glycol) diacrylate (PEGDA), alginate, poly(ε-caprolactone) (PCL), oligo(poly-ethylene glycol) fumarate (OPF), poly( N -isopropylacrylamide) (PNIPAAm), poly(hydroxyethyl methacrylate) [p(HEMA)] and others ( Fig. 16.1 ), the use of hyaluronic acid-based (HA) hydrogels has received significant attention. HA is a non-sulfated linear polysaccharide of (1-β-4) d -glucuronic acid and (1-β-3) N -acetyl- d -glucoasamine, and is a major constituent of the ECM during early embryogenesis, as well as in adult connective, epithelial and neuronal tissue . HA hydrogels are capable of storing, propagating and differentiating stem cells . Cross-linking HA hydrogels allows for the manipulation of their mechanical properties such as rigidity and elasticity , whereas the application of hyaluronidase permits rapid dissolution of the hydrogels. These cross-linked 3D scaffolds can be coated with various adhesion molecules and have been used to preserve pluripotent stem cells for 3 weeks . Different purposes dictating different adhesion molecules and motifs, incorporation or coating of scaffolds with RGD, YIGSR or IKVAV peptides or their combinations have been reported . Similarly, inductive growth factors such as transforming growth factor-β (TGF-β), hepatocyte growth factor (HGF), or basic fibroblast growth factor (bFGF) incorporation into hydrogels has been shown to improve regeneration of muscle and bone in vivo . The size of pores, which is a function of the degree of cross-linking, affects stem cell behavior: pores of 30–60 μm allow for more rapid cell growth than pores of 60–130 μm . The ability to incorporate stem cells, adhesion molecules and factors responsible for the maintenance and regulation of quiescence or proliferation of these cells provides this bioartificial stem cell carrier/niche with substantial flexibility to enable regulation of stem cell fate. Real-time control over the properties of scaffolds can be achieved by using switchable coatings sensitive to physical parameters such as temperature, light, pH and electromagnetic field to regulate protein-surface and cell-surface interactions.

Fabrication Techniques
Three techniques for the fabrication of hydrogels are typically used. These are chemical cross-linking, photo-cross-linking and sol-gel synthesis. In addition to these major methods, some hydrogels are formed through cryogelation, spinning, bioprinting, centrifugal casting or a microfabrication process.
Chemical Cross-linking
The most common form of gelling polymers to form hydrogels is through the addition of a bifunctional chemical compound that will react with functional groups on the polymer backbones to cross-link them. This usually involves reactions with carboxyl, amine or thiol groups that branch off the main chain of the polymer. Sometimes heat or agitation is required for the cross-linking to occur. Depending on the concentration of cross-linker and gel precursors in the solution the gelling can take anything from minutes to hours to complete . Some systems require alternate forms of cross-linking, such as alginate, which is cross-linked by the addition of a divalent cation, most commonly Ca 2+ . Chemical cross-links are also most common because the chemicals are typically non-toxic, which is necessary for both in vitro and in vivo applications, and allow for a much greater control over the location of the linkages.
Photo-cross-linking
Photo-cross-linking is typically achieved by the addition of an ultraviolet (UV)-sensitive photoinitiator, although some initiators are also susceptible to visible light . The initiator begins cross-linking the hydrogel through a radical polymerization of double bonds in the hydrogel precursors. This cross-linking technique requires the addition of double bonds to the gel backbone in order to function properly. The advantages of photo-cross-linking over chemical cross-linking are that the reaction usually completes on a shorter time-scale, seconds to minutes, and tends to release almost no heat, whereas chemical cross-linking may require heating to proceed . However, radical polymerization tends to be more difficult to control. As a more energetic reactive species, radicals can react at unpredictable places and do not necessarily target the same atom every time. This leads to a more random hydrogel that can have different properties at different locations throughout the gel.
Methacrylated HA (HA-MA) was recently employed in research on cutaneous and corneal wound healing , embryonic stem cell expansion , and drug and growth factor delivery . In addition, photo-cross-linked HA-MA provided a 3D microenvironment suitable for mesenchymal stem cells (MSCs) to differentiate into a chondrogenic phenotype .
Sol-gel Synthesis
Sol-gel synthesis uses a simple phase transition to convert the hydrogel precursors to a stable gel form. This effect is usually pH or temperature dependent and can be very useful for drug delivery applications where an injected hydrogel solution mixed with a drug molecule transitions at or below body temperature to form a gel . The drug molecule then diffuses slowly out of the gel in a time-controlled manner based on the degree of physical cross-linking. Cells can also be encapsulated in this manner, and remain viable .
Cryogelation
This synthesis technique, like sol-gel synthesis, also creates a physically cross-linked hydrogel. When the gel precursors are at room temperature, they form a solution, although a potentially viscous one. When frozen, the ice crystals that form tend to aggregate the polymer chains together. Upon thawing, the chains form non-covalent bonds resulting in a hydrogel . This technique can be used to store molecules or even cells for extended periods. However, the polymers for which this synthesis technique is possible tend to be synthetic and thus do not contain many adhesive sites for cells. This problem is typically overcome by the addition of some other polymer such as collagen or chitosan that contains adhesion sites for the cells .
Spinning
Spinning is a technique where a hydrogel precursor solution is extruded through a needle to form microscaled or nanoscaled fibers. This is typically achieved using electrospinning without a cross-linking agent. In electrospinning, a large voltage is applied to the needle as the solution is pushed through with a syringe pump. As the charged solution exits the needle, a “taylor cone” is formed where the electrostatic repulsion is high enough that a very fine stream of solution erupts from the needle. During flight, the solvent dries out and the charge moves to the surface of the forming fiber. This causes the fiber to whip around owing to the electrostatic repulsion felt over small bends ( Fig. 16.2 D). The fiber is finally collected on a grounded plate , or for aligned fibers, separated collecting plates or a spinning mandrel or disc . In addition to electrospinning, hydrodynamic spinning is a potential fabrication technique . In hydrodynamic spinning, a similar extrusion process is used; however, the addition of a cross-linker is required. The diameter of the fibers formed is determined by the flow rate through the apparatus, and hollow fibers can be formed from the combination of three concentric streams .

Bioprinting
The technique of bioprinting consists of two components. First, cell aggregates, cellularized sECM hydrogels or cell-seeded microspheres comprise the “bioink.” The cell-free polymers that provide the substratum for the bioink are the “biopaper.” Bioprinting consists of stepwise assembly of bioink and biopaper components into an organ-appropriate 3D architecture using a three-axis printer . For example, vascular networks can be printed by a “scaffold-free” process involving automated deposition of sausage-like cell aggregates and agarose tubes . In each case, computer-assisted design is used to guide the deposition of cells in geometries that recapitulate the structure of the target tissue or organ . After printing, the engineered construct is allowed to mature and gain functionality in a bioreactor or in vivo environment .
Centrifugal Casting
Living polymers, such as the sECMs described below, offer the unique opportunity of forming a wide variety of tubular structures in the body, including potentially kidney nephrons, by centrifugal casting . This technique was first validated by sandwiching a layer of fluorescently labeled endothelial cells between two layers of an in situ cross-linkable sECM by axial centrifugation at approximately 11 × g . After centrifugally coating the inside of a Dacron vascular prosthesis with the sECM, a cell suspension was applied and centrifugally cast as the gel cross-linked during centrifugation. The heavier cells were entrapped between the two layers, remained viable and remodeled the cross-linked HA–gelatin sECM. Cells in the sECM suspension can also be applied to laser-machined micropores of a sheet of small intestinal submucosa (SIS), which affords a robust construct that retains the cell-seeded hydrogel and permits rapid biofabrication of a tubular tissue in a bioreactor-free fashion .
Microfabrication
Complex patterns can also be generated in hydrogels using various microfabrication techniques, typically by reshaping a preformed hydrogel. Microspheres of hydrogels can be formed through the use of emulsification polymerization . Here a mechanical agitation of the hydrogel precursors in an organic phase causes microspheres to form which can then be gelled through a variety of cross-linking methods ( Fig. 16.2 C). Size can be controlled through the addition of surfactant molecules and the degree of agitation .
Photolithography is another useful technique for the fabrication of complex patterns . A thin layer of hydrogel precursor is applied to a substrate. Then a photomask with the desired pattern is placed over the hydrogel and the entire assembly is exposed to UV light, which causes gelation of the pattern ( Fig. 16.2 A). The remaining hydrogel can then be washed away. Photolithography is capable of producing complex features on a submicrometer to millimeter scale. However, photolithography is essentially a 2D approach and multiple steps may be required to achieve a 3D structure.
Microfluidics can also be used to create hydrogels . Here, small channels are formed in a polymer such as poly(dimethyl siloxane) (PDMS) by molding it from a master. The device is then sealed by placing the PDMS mold onto a piece of glass, or a stronger seal can be formed by air plasma treatment of the PDMS before inversion on glass . Hydrogel precursors can be pumped in through multiple inlets and by controlling the flow rate and design of the channels, various morphologies can be generated ( Fig. 16.2 E). Typically, rods and spheres are formed but hybrid particles can also be created where each side has a different composition . In addition, microfluidics can be combined with photolithography to capture cells in a particular location by cross-linking the surrounding hydrogel .
Finally, micromolding is a simple technique for the fabrication of hydrogels with micrometer-scale features . Micromolding is based on a technique called soft lithography, where a PDMS stamp is molded from a prefabricated silicon wafer ( Fig. 16.2 B). Hydrogel precursors are molded with the PDMS stamp and subsequently gelled by the addition of a cross-linking agent through the mold, by irradiation for photopolymerization or by a sol-gel transition. Micromolding is a fast and efficient technique for generating 3D structures and can support features down to the nanoscale.
Common Hydrogel Materials
The proper materials selection is important when designing a hydrogel for a specific application. Depending on the use, a hydrogel needs to exhibit different properties in vivo. There are three general classes of biocompatibility for both natural and synthetic hydrogels. There are biomimetic hydrogels that emulate a naturally occurring component of the target implant site, biodegradable hydrogels that slowly dissolve in vivo to release drug molecules or cells, and bioinert hydrogels that have very little interaction with the biological tissue. Each type of interaction can be relevant for a particular application.
Biomimetic Hydrogels
These materials tend to have very similar properties to the implant site, whether they are natural or synthetic, and are usually able to integrate seamlessly with the actual tissue. Collagen/gelatin and HA are two natural compounds that are major components of the ECM. Other ECM proteins such as fibrin, fibronectin and laminin have also been used to fabricate hydrogels . Synthetic hydrogels can be combined with other naturally occurring polymers to create biomimetic implants, such as those used for bone repair , which must be stronger than a natural material on its own.
Biodegradable Hydrogels
Most natural hydrogel materials are biodegradable by enzymes that are naturally produced by the body. Among synthetic hydrogels, those made from polymers of amino acids, such as poly(lactic acid) (PLA) and poly(glycolic acid) (PGA), are easily biodegradable. Others, such as PCL and OPF, are polyesters that can be slowly degraded through hydrolysis. Biodegradable hydrogels can be tuned to degrade at different rates by varying cross-linker concentration and through the addition of other monomers to form block copolymers that may degrade at a faster or slower rate .
Bioinert Hydrogels
The last class of hydrogels is bioinert hydrogels. These elicit essentially no immune or inflammatory responses when implanted. Poly(ethylene glycol) (PEG) and poly(vinyl alcohol) (PVA) are two examples of synthetic bioinert hydrogels ( Fig. 16.1 ). PEG and PVA are very hydrophilic and offer very few attachment sites for cells. These materials can be used to help isolate encapsulated cells from an immune response or serve as long-term repositories for drug delivery . They are also very stable in water because the backbone of PVA is all carbons, and that of PEG is polyether. PEG is commonly used as a surface treatment for other implants because of its ability to be conjugated to other functional groups through terminal alcohol domains.
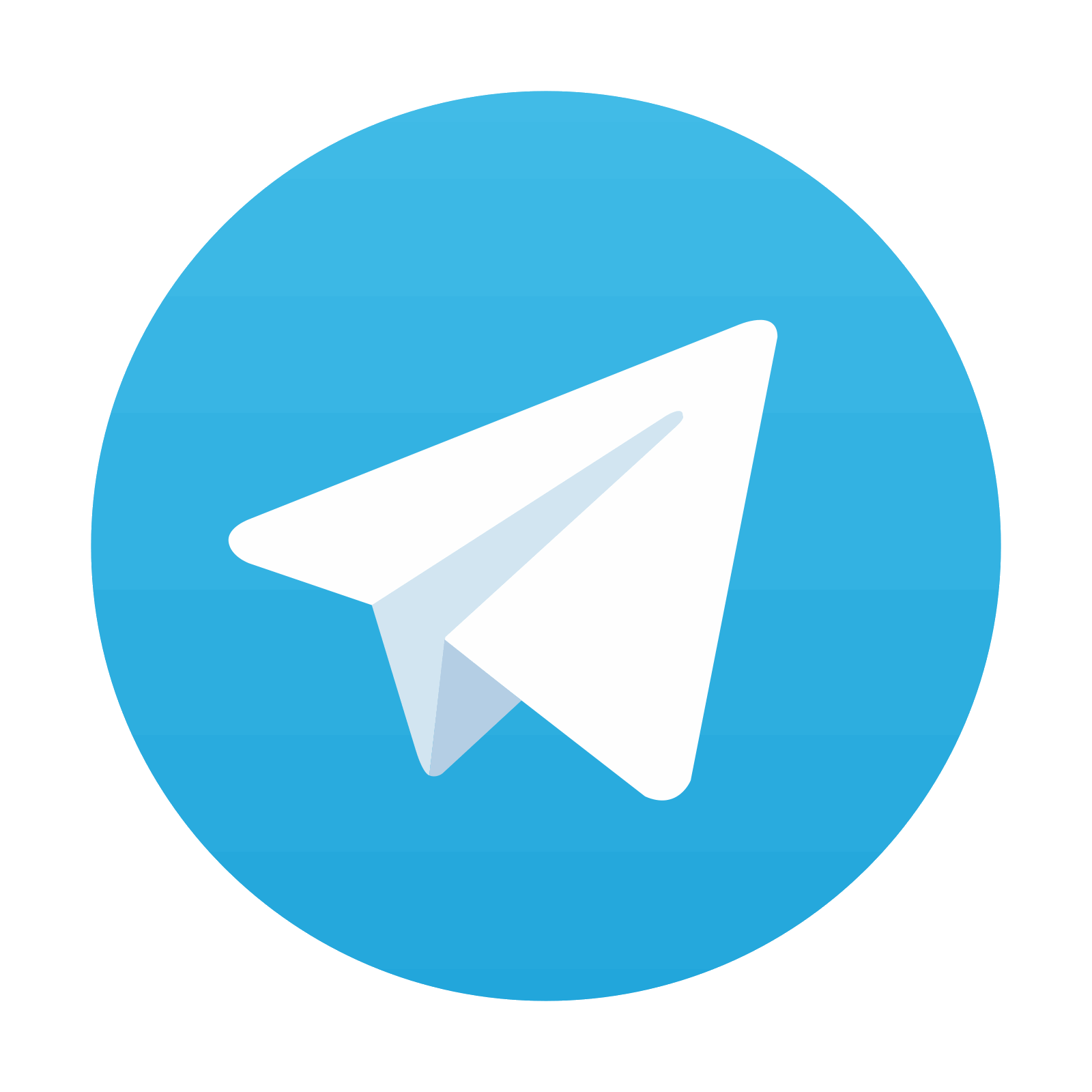
Stay updated, free articles. Join our Telegram channel
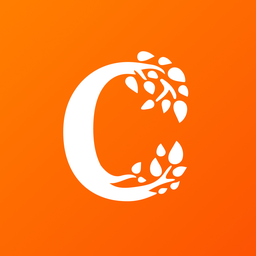
Full access? Get Clinical Tree
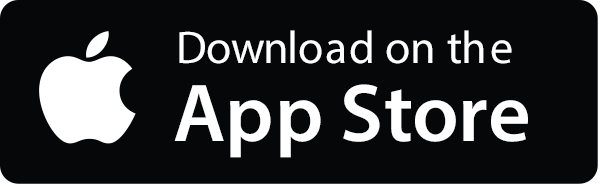
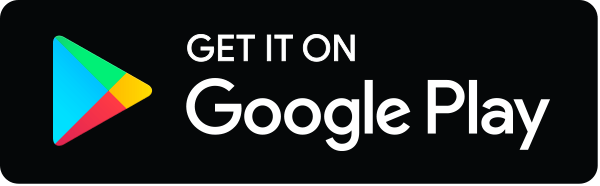