Fig. 8.1
Etiology of PSC
Three ulcerative colitis (UC) susceptibility loci associated with PSC, harboring the putative candidate genes REL, IL2, and CARD9, have been identified [6]. A study reported 12 significant genome-wide associations outside the HLA region, nine of which were new, increasing the number of known PSC risk loci to 16. Despite comorbidity with IBD in 72% of the included cases, six of the 12 loci showed significantly stronger associations with PSC than with IBD, suggesting overlapping, yet distinct, genetic mechanisms for the two diseases [7].
Translocation of microbial flora across the gut is one hypothesized mechanism for the development of PSC [8]. Small intestinal bacterial overgrowth and the introduction of bacterial antigens into the portal circulation can cause pericholangitis in animal models [9–11]. However, studies in humans have suggested that portal venous bacteremia is uncommon in UC [12]. Although some antibiotics have been shown to reduce serum alkaline phosphatase levels and Mayo risk scores, the long-term effects of antibiotics on PSC progression are unclear [13, 14]. Growing interest in the relationship between the human microbiome and chronic disease will undoubtedly lead to studies in patients with PSC.
Normally, biliary epithelial cells are exposed to common intestinal pathogen-associated molecular patterns, such as lipopolysaccharide and lipoteichoic acid. However, exposure to lipopolysaccharide may disrupt tight junctions in colonic and biliary epithelial cells, through Toll-like receptor (TLR)4-dependent mechanisms [14, 15]. Alteration of such barriers can expose cholangiocytes to a variety of substances, such as bile acids, that could promote injury and inflammation. Disruption of cholangiocyte tight junctions is an important step in the development of PSC in animal models [16, 17]. For example, mice with altered cholangiocyte tight junctions exhibit leakage of bile acids into the portal tract. This leads to an inflammatory response involving CD8+ and CD4+ T cells and upregulation of tumor necrosis factor (TNF)-α, transforming growth factor-β1, and interleukin (IL)1β. This inflammatory infiltrate causes myofibroblast activation and fibrosis [16].
The pathogenesis of PSC has been examined from the standpoint of PSC-IBD. Translocation of the microbial flora across an inflamed, permeable gut with subsequent activation of the immune system and inflammation of the biliary tree is another hypothesized mechanism for the development of PSC (Fig. 8.2). It appears that the α4β7+CCR9+CD8+ T cells that infiltrate the liver in PSC are primed by dendritic cells in the intestine. Activated intestinal lymphocytes enter the enterohepatic circulation and persist as memory cells that cause hepatic inflammation. Chemokines and adhesion molecules shared by the intestine and liver could contribute to immune cell binding at both sites [18].


Fig. 8.2
Mechanism and treatment for immune system
The observations that PSC can develop after colectomy [19] and that IBD can develop after liver transplantation have caused some investigators to suggest that aberrant homing of lymphocytes between the intestine and liver could be involved in the pathogenesis of PSC [20]. Three studies indirectly supporting this theory have been published. Patients who received a liver transplant had lower clinical and histological IBD activities than those of the non-transplant group [21]. Marelli et al. [22] reported that progressive PSC requiring liver transplantation is associated with a milder course of UC, including reduced disease activity and use of steroids, azathioprine, and surgery. Navaneethan et al. [23] reported that severe, progressive PSC requiring liver transplantation appeared to reduce the disease activity of UC and the need for a colectomy. However, these theories cannot explain why only 2–7.5% of IBD patients develop PSC [24], whereas PSC is strongly associated with development of IBD, or why Crohn’s disease is less associated with PSC. It is also unclear why immunosuppression does not improve PSC.
The involvement of adhesion molecules in lymphocyte recruitment to the liver is emerging as an important step in the pathogenesis of PSC. Inflammatory mediators appear to upregulate various adhesion molecules during the development of PSC, including intercellular adhesion molecules, vascular cell adhesion molecule-1 (VCAM-1) [25], and mucosal addressin cellular adhesion molecule 1 (MAdCAM-1) [26] (Fig. 8.2). Typically, MAdCAM-1 is expressed in the mucosal vessels of the intestine. However, under conditions of inflammation, it can be expressed by the hepatic endothelium [27]. Patients with PSC have also been observed to have altered expression of chemokines, such as CCL25, CCL28, CXCL12, and CXCL16. Upregulation of CCL25 and CCL28 leads to activation of α4β7 integrins, which increase lymphocyte binding to MAdCAM-1. CCL28 also appears to activate α4β1 integrin and to increase its adhesion to VCAM-1, which is primarily expressed in the portal and sinusoidal endothelial cells of diseased liver, so it might not be a specific feature of PSC [28].
8.3 Treatment for PSC
At this time, there is no beneficial medical therapy for patients with PSC, and liver transplantation is the only potentially curative option for patients with PSC. Therapies for PSC can be classified roughly into two categories: regulation of immunomechanisms and bile acids. The former therapies mainly regulate the interaction between adhesion molecules and lymphocyte recruitment to the liver (Fig. 8.2). The latter alter the composition of the bile acid pool and reduce the total serum bile acid pool (Fig. 8.3). Recent trends and clinical trials of treatment for PSC are reviewed in Ali’s paper [29].


Fig. 8.3
Treatment for PSC regulation of bile acid
8.3.1 Regulation of Immunomechanisms
Several agents including immunosuppressants and immunomodulators, such as azathioprine [30], budesonide, methotrexate [31], prednisolone, and tacrolimus, have been evaluated in PSC patients but failed to show positive results. VCAM-1 and MAdCAM-1 are expressed in the mucosal vessels of the intestine and hepatic endothelium in PSC patients [26, 27] (Fig. 8.2). Vascular adhesion protein 1 (VAP-1) has been found to induce the expression of MAdCAM-1 in the hepatic endothelial cells of human liver tissue [25]. Targeting VAP-1 and MAdCAM-1 could be beneficial in patients with PSC. A monoclonal antibody against α4β7 and a VAP-1 blocking agent have been investigated [32]. Liver-infiltrating T cells recruited by aberrant expression of the gut-specific chemokine CCL25 activate α4β7 binding to MAdCAM-1 on the hepatic endothelium. Targeting the CCL25-MAdCAM-1 axis could have a beneficial effect [33].
Several animal experiments have demonstrated a link between the gut microbiota and development of PSC [34]. Induction of small bowel bacterial overgrowth by ligation of the jejunum in rats resulted in the development of hepatic lesions compatible with PSC. Daily treatment with antibiotics resulted in significant improvement of these lesions, suggesting that modification of the gut microbiota could be of therapeutic benefit at least in a selected group of PSC patients. Vancomycin, metronidazole, and minocycline have been evaluated in clinical trials in patients with PSC [35, 36]. The use of these antibiotics resulted in a significant reduction in serum alkaline phosphatase (ALP), an important surrogate marker in PSC. Thus, antibiotic therapy for PSC patients seems to be a promising tool in the treatment of PSC.
8.3.2 Regulation of Bile Acids
The synthetic bile acid ursodeoxycholic acid (UDCA) is the 7-β-epimer of chenodeoxycholic acid (CDCA). Because it has been shown to improve liver biochemistry, UDCA has been used frequently for therapy in PSC. However, there is no clear evidence that UDCA contributes to improvements in patient survival.
An abnormal composition of the bile acid pool is thought to play a key role in the pathogenesis and progression of PSC [37] (Fig. 8.3). This hypothesis was derived from several animal [38] and human studies. PSC-like lesions occur in mice devoid of the canalicular transporter Mdr2, which mediates biliary excretion of phospholipids that normally form mixed micelles with bile acids, thus protecting the liver against the detergent effects of bile acids [39]. Bile acid toxicity to the biliary epithelium could result from decreased biliary HCO3 secretion [40]. The bile salt-sensing receptor TGR5 plays a key role in the regulation of HCO3 secretion, and TGR5 was identified as a likely disease-susceptibility gene in a large genome-wide study of PSC [41].
Animal and in vitro studies have also suggested that UDCA may have a role as a chemopreventive agent in the prevention of colorectal neoplasia. Several mechanisms in which UDCA may act to prevent colorectal cancer have been proposed, including downregulation of cyclooxygenase-2 expression; prevention of carcinogen-induced changes in protein C; inhibition of cell proliferation by suppressing epidermal growth factor receptor, which is typically activated by deoxycholic acid (DCA); and alteration of the bile acid milieu to reduce secondary bile acid levels [42–46]. Lower doses of UDCA may have a protective role because of its potential antiapoptotic effect. For example, exposing human colon cancer cell lines to UDCA can decrease DCA-induced apoptosis [47].
Multiple human studies also have investigated the role of UDCA as a chemopreventive agent, but the results are conflicting. Two clinical studies suggested that UDCA reduces the incidence of colorectal neoplasia in patients with UC and PSC [48, 49]. Tung et al. [48] performed a retrospective review of 59 patients and found that UDCA was associated with a significant reduction in the odds ratio for colonic dysplasia development. However, after excluding cases of indefinite dysplasia, a multivariate analysis did not reveal a statistically significant association with UDCA. A secondary analysis revealed a significant association between UDCA use and the development of high-grade dysplasia only. Furthermore, in this study, the control group had a high proportion of dysplasia (72%), and 50% of those who received UDCA went on to develop dysplasia. Compared with those who did not receive UDCA, the UDCA-treated group had a shorter duration of colitis and were older at the time of diagnosis of colitis. A third study indicated that the times of cancer and dysplasia development were the same, regardless of whether subjects received UDCA [50]. Consequently, it remains unclear in clinical practice whether patients with PSC and UC should be started on UDCA for the prevention of colonic neoplasia.
Treatment with high-dose UDCA, compared with placebo, was associated with an increased rate of serious clinical events. A multicenter, placebo-controlled trial investigated the use of UDCA 28–30 mg/kg/day in patients with PSC. That study revealed an improvement in liver biochemistry, but no improvement in survival, and a higher rate of adverse events [51, 52], as well as the development of colon cancer [53] in the UDCA group. Sinakos et al. investigated the serum bile acid composition in patients in the high-dose UDCA arm and compared it with that of control group patients [54]. Long-term use of high-dose UDCA is associated with an increased risk of colorectal neoplasia in patients with UC and PSC. In total, 56 subjects were followed for a total of 235 patient years. Patients who received high-dose UDCA had a significantly higher risk of developing colorectal neoplasia (dysplasia and cancer) during the study compared with those who received placebo (hazard ratio = 4.44, 95% confidence interval = 1.30–20.10, P = 0.02). They concluded that bile acids may have a role in colon carcinogenesis, particularly the secondary bile acids (lithocholic acid (LCA) and DCA) [55–57]. In in vitro studies, prolonged exposure to high bile acid concentrations has been suggested to play a role in gastrointestinal cancers, leading to oxidative stress, selection of apoptosis-resistant cells, and replication of unrepaired damaged DNA [57, 58]. They reported a statistically significant increase in serum UDCA and LCA levels in the treatment group, compared with the placebo group, and an expansion of the total serum bile acid pool without significant changes in CA, DCA, or CDCA levels [54]. It is expected that a proportion of the UDCA pool would be metabolized by bacterial flora into other bile acids. UDCA can be metabolized into both LCA and CDCA by intestinal flora. CDCA is typically metabolized to LCA by bacterial flora. Both CDCA and LCA have been shown to stimulate in vitro cell invasion in a dose-dependent manner [59, 60]. Furthermore, physiological levels of CDCA and DCA can increase colon adenoma cell proliferation and reduce apoptosis [61]. The American Association for the Study of Liver Disease (AASLD) recommends against the use of UDCA in PSC patients [62].
NorUDCA is a C23 homolog of UDCA that is currently being evaluated in a phase II randomized clinical trial in patients with PSC. In a rodent model of cholestasis, the administration of norUDCA to Mdr2 knockout mice improved sclerosing cholangitis, possibly by altering the composition of the bile acid pool by displacing the toxic bile acids and increasing the hydrophilicity of the bile acids [63]. In a more recent animal model of cholestasis, norUDCA, compared with UDCA, significantly improved indices of liver injury in common bile duct-ligated mice [64].
The farnesoid X receptors (FXRs) are a group of nuclear hormone receptors expressed at high levels in tissues involved in bile acid metabolism, such as the liver, intestine, and kidney [65]. Bile acids have been identified as natural ligands of FXRs [66, 67]. FXRs play a key role in bile acid homeostasis by regulating genes involved in bile acid synthesis, secretion, conjugation, transportation, absorption, and detoxification [68–72] (Fig. 8.3). An important target of the FXRs is the gene encoding cholesterol 7α hydroxylase (CYP7A1), the rate-limiting enzyme in bile acid biosynthesis. When bound to bile acids, FXRs repress this gene [66]. Moreover, the expression of an important transport protein (cytosolic intestinal bile acid-binding protein) [73], located in the intestines, is increased as a result of activation of the FXRs [66, 74]. This protein is believed to play a key role in the regulation of the enterohepatic circulation [66, 74]. In addition to their role in bile acid homeostasis, FXRs have been found to regulate liver regeneration in liver injury [75–80].
Obeticholic acid (OCA, INT-747), a 6-ethyl derivative of the natural human bile acid CDCA, is a first-in-class selective FXR agonist, with ~100-fold greater FXR agonistic activity than that of CDCA [81–83]. In a male Wistar rat model of cholestasis, OCA protected hepatocytes against the deleterious effects caused by administration of LCA [83]. In another animal model, the administration of OCA reduced liver fibrosis and indices of hepatic damage in bile duct-ligated rats [84]. These results suggest that FXR agonists could be of therapeutic benefit in patients with cholestatic liver diseases. The safety and efficacy of OCA has been evaluated in two randomized clinical trials in patients with primary biliary cirrhosis (PBC) with promising results [85, 86]. The administration of OCA to PBC patients resulted in a significant reduction of serum ALP, an important surrogate marker in PBC [85, 86]. One important adverse event was pruritus, which was affected by OCA in a dose-dependent manner and led to discontinuation of the drug in 38% of PBC patients [85, 86].
The apical sodium-dependent bile acid transporter (ASBT), also known as the ileal bile acid transporter, is expressed predominantly in the distal ileal tissue and plays a key role in the reabsorption of bile acids from the lumen of the small intestine, which is critical for the enterohepatic circulation of the bile acids [87]. Normally, ~95% of secreted bile acids are reabsorbed from the intestine into the portal circulation and back to the liver [88]. Under this biological rationale, interrupting the enterohepatic circulation could result in a decreased bile acid load on the liver, which, in turn, could be of potential therapeutic benefit in patients with PSC (Fig. 8.3). The safety and efficacy of an ASBT inhibitor is being evaluated in patients with PSC.
8.4 Conclusion
Here, we summarized the etiology and treatment of PSC with regard to bile acids. Genetic backgrounds and immune mechanisms for PSC are becoming clarified gradually. New therapeutic strategies have also been trialed. Further studies are necessary to clarify the etiology of PSC.
Acknowledgments
This work was supported by Health Labor Science Research Grants from Research on Measures for Intractable Diseases, the Intractable Hepato-biliary Diseases Study Group in Japan.
References
1.
Lazaridis KN, Wiesner RH, Porayko MK, Ludwig J, LaRusso NF. Primary sclerosing cholangitis. In: Schiff ER, Sorrel MF, Maddrey WC, editors. Schiff’s disease of the liver. English ed. Philadelphia: Lippincott-Raven; 1999. p. 649–78.
2.
Lindor KD, LaRusso NF. Primary sclerosing cholangitis. In: Schiff L, Schiff ER, editors. Schiff’s disease of the liver. 9th ed. Philadelphia: JB Lippincott; 2003. p. 673–84.
4.
Dew MJ, van Berge Henegouwen GP, Huybregts AW, Allan RN. Hepatotoxic effect of bile acids in inflammatory bowel disease. Gastroenterology. 1980;78:1393–401.PubMed
5.
Pollheimer MJ, Halilbasic E, Fickert P, Trauner M. Pathogenesis of primary sclerosing cholangitis. Best practice & research. Clin Gastroenterol. 2011;25:727–39. doi:10.1016/j.bpg.2011.10.009.
6.
Janse M, Lamberts LE, Franke L, Raychaudhuri S, Ellinghaus E, Muri Boberg K, et al. Three ulcerative colitis susceptibility loci are associated with primary sclerosing cholangitis and indicate a role for IL2, REL, and CARD9. Hepatology. 2011;53:1977–85. doi:10.1002/hep.24307.CrossRefPubMedPubMedCentral
7.
Liu JZ, Hov JR, Folseraas T, Ellinghaus E, Rushbrook SM, Doncheva NT, et al. Dense genotyping of immune-related disease regions identifies nine new risk loci for primary sclerosing cholangitis. Nat Genet. 2013;45:670–5. doi:10.1038/ng.2616.CrossRefPubMedPubMedCentral
8.
O’Mahony CA, Vierling JM. Etiopathogenesis of primary sclerosing cholangitis. Semin Liver Dis. 2006;26:3–21. doi:10.1055/s-2006-933559.CrossRefPubMed
9.
Lichtman SN, Sartor RB. Hepatobiliary injury associated with experimental small-bowel bacterial overgrowth in rats. Immunol Res. 1991;10:528–31. doi:10.1007/BF02919752.CrossRefPubMed
10.
Yamada S, Ishii M, Liang LS, Yamamoto T, Toyota T. Small duct cholangitis induced by N-formyl L-methionine L-leucine L-tyrosine in rats. J Gastroenterol. 1994;29:631–6. doi:10.1007/BF02365447.CrossRefPubMed
11.
Pollheimer MJ, Trauner M, Fickert P. Will we ever model PSC? – “it’s hard to be a PSC model”. Clin Res Hepatol Gastroenterol. 2011;35:792–804. doi:10.1016/j.clinre.2011.04.014.CrossRefPubMed
12.
Palmer KR, Duerden BI, Holdsworth CD. Bacteriological and endotoxin studies in cases of ulcerative colitis submitted to surgery. Gut. 1980;21:851–4. doi:10.1136/gut.21.10.851.CrossRefPubMedPubMedCentral
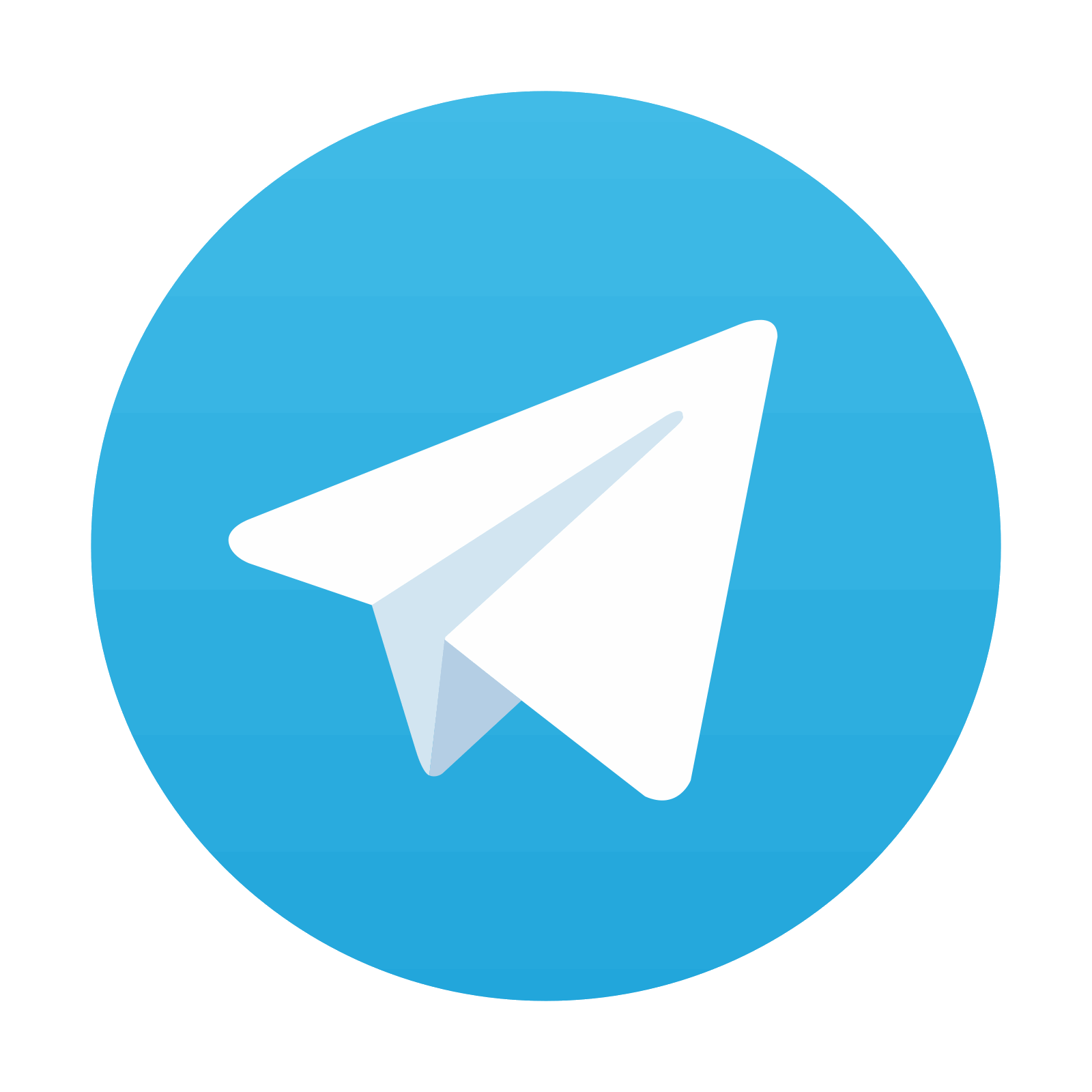
Stay updated, free articles. Join our Telegram channel
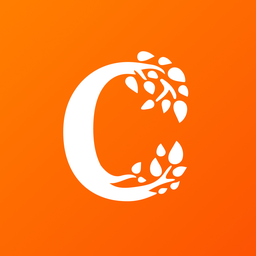
Full access? Get Clinical Tree
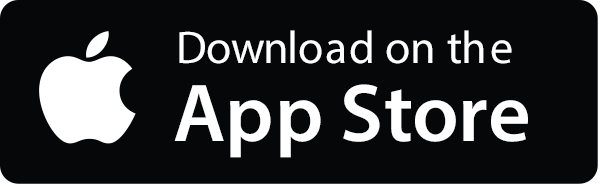
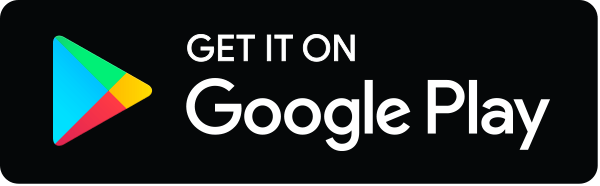