Oral agents
Intravenous agents
Most common agents
Ferrous fumarate
Ferric gluconate
Ferrous gluconate
Ferumoxytol
Ferrous sulfate
Iron sucrose
Polysaccharide-iron complex
Side effects
Gastrointestinal (metallic taste, flatulence, constipation, diarrhea, abdominal pain, green stool, etc.)
Hypersensitivity reaction (mostly at injection site, rarely anaphylaxis)
Itching
Dizziness
Headaches
Itching
Comparison
Inexpensive
Costly
Frequent dosing
Infrequent dosing
Poor compliance
Better compliance
Safer
More efficacious and reliable
Currently, one of the major limitations to the use of IV iron is the theoretical potential for exacerbating bacterial infections and/or bacteremia. This hypothetical risk stems from the fact that iron excess in vitro catalyzes multiple essential steps in bacterial cell metabolism, growth, DNA/RNA replication, and protection from free radical killing, as with inhibiting the immune system [42–44]. States of iron overload, either primary or secondary, have been associated with higher rates of bacterial infections and bacteremia [45]. Recent data looking directly at the impact of iron infusion on the infectious rates of dialysis patients suggest it may be safe in the setting of active bacterial infection [46, 47]. Nevertheless, the data compiled thus far is retrospective and correlational and no randomized controlled data exist looking directly at the impact of infusing IV iron in the setting of culture-proven bacteremia, in patient who may otherwise benefit from iron supplementation.
Pharmaceutical Therapy
In its original phase III trial in 1989, recombinant human erythropoietin (rHuEPO) demonstrated an impressive ability to improve hematocrit, raising hematocrit from 22.3 to 35% in 12 weeks in greater than 97% of hemodialysis patients, eliminating the need for transfusions, and in patients with iron overload, decreased serum ferritin by nearly 40% [48]. Consequently, there was a dramatic increase in the average hemoglobin of all dialysis patients. Transfusions decreased by greater than two times from 1992 to 2005, with most of the drop observed in the first 5 years after the introduction of rHuEPO, nearly eliminating severe anemia as a major cause of death in ESRD patients [49]. Encouraged by these data, physicians began using supratherapeutic doses, in an attempt to normalize hemoglobin, the consequences of which are discussed later. Currently, there are multiple formulations of ESAs (Table 6.2).
Table 6.2
Comparison of different ESAs
Formulation | Half-life (h) | Dosing (days) | Modification to epoetin alfa | Disadvantage |
---|---|---|---|---|
Epoetin Alfa | 7–8 | 2–3 | – | Frequent dosing |
Darbepoetin | 25 | 7–14 | Hyperglycosylated | |
C.E.R.A. | 130 | 14–28 | Pegylation | |
Peginesatide | 25 | 28 | NOT epo-based | Hypersensitivity |
The history of the development of rHuEPO deserves some focus, as the process was unique in its time. In 1985, after isolating and cloning the gene for human erythropoietin, researchers were able to introduce this gene into Chinese hamster ovary cells , and consequently produce biologically active human erythropoietin [50]. The gene encodes a glycoprotein consisting of a 165-amino-acid sequence with a glycosylated portion essential for its safeguard against enzymatic degradation [51]. In 1989, epoetin alfa was created and separately marketed by the pharmaceutical companies Johnson & Johnson and Amgen, who labeled their products Procrit™ and Epogen™ , respectively. In 1991, epoetin beta was introduced, and while having an identical amino acid sequence to epoetin alfa, its carbohydrate moiety differs in configuration and consequently its half-life [52, 53]. Despite its longer half-life, it has not gained widespread use in the United States. Pure red cell aplasia is an uncommon disorder associated with the absence of red cell precursors, low reticulocyte count, and severe anemia. This disease is generally idiopathic but in a few rare situations, it has been associated with the formation of autoantibodies to erythropoietin in the setting of ESA use. The vast majority of these cases were in association with epoetin beta, and the European formulation of epoetin alfa, Eprex™ manufactured by Janssen-Cilag.
One widely accepted, extended release formulation is darbepoetin alfa, which is a hyperglycosylated epoetin alfa [54, 55]. In fact, with its 25.3 h half-life and once-weekly schedule, it has become the predominant ESA for the treatment of CKD patients in the outpatient setting [56]. Darbepoetin alfa is marketed by Amgen under the trade name Aranesp™.
Methoxy polyethylene glycol-epoetin beta, a continuous erythropoietin receptor activator (Micera™ ), was created with an even longer half-life of over 130 h, by the adding polyethylene glycol to epoetin beta [57]. With a once-monthly dosing, Micera™ can have a significant cost reduction of 59% [58].
All ESAs mentioned thus far have relied on adding varying types of carbohydrate molecules to the parent peptide in order to deliver the original erythropoietin molecule in a stable vehicle. Peginesatide, developed in March of 2012, is a peptide ESA that has no sequence homology with erythropoietin but a similar intracellular signaling cascade [59, 60]. Its efficacy was confirmed in CKD patients in the PEARL 1 and 2 trials, but these trials demonstrated significantly higher cardiovascular end points as compared to darbepoetin, consequently this medication was approved only for use in dialysis patients [61]. Its lack of sequence homology with erythropoietin made it an ideal agent in the setting of anemia due to erythropoietin-induced antibody-mediated pure red cell aplasia, and in fact, peginesatide was used on 14 of these patients with positive results [62]. Unfortunately, post-market safety surveillance indicated a high rate of hypersensitivity reactions with the initial administration of the drug, including anaphylaxis and death. In 2013, peginesatide was withdrawn from the market [63].
With all the above available erythropoiesis-stimulating agents, as well as biosimilar formulations, the principal ESAs in use in the U.S. remains epoetin alfa and its hyperglycosylated counterpart darbepoetin alfa.
There remains an ongoing debate as to which target hemoglobin should these agents be used to achieve. There is no doubt that these agents can reliably and effectively raise hemoglobin, the open question is; where is the balance between clinical benefit and safety. If, for example, raising hemoglobin enables the patient to avoid the ischemic cardiac penumbra and the side effects of blood transfusion, then in theory, normalizing hemoglobin should be the goal. Indeed, this was the predominant belief in the late 1990s and early 2000s.
But, initial doubts about normalizing hemoglobin were the first cast in 1998 with the Normal Hematocrit Study [64]. This trial looked at 1233 hemodialysis patients with cardiac disease who were randomized to either partial or complete correction of hemoglobin, with epoetin alfa. Surprising at the time, the study was terminated early due to safety signals in the normalized hemoglobin group. While there were more events in the normal hematocrit group (N = 202 vs. 164 in the partial correction group), the difference was not clinically significant. However, this was the first trial to suggest harm at higher hemoglobin targets. Although this study was conducted on dialysis patients, one may, inaccurately, be tempted to generalize these results to the patient with CKD.
The first direct evidence that higher hemoglobin targets may be detrimental to CKD patients came from the CREATE and CHOIR trials [65, 66]. In the CREATE trial, 603 CKD patients were randomized to either a target hemoglobin of 10.5–11.5 g/dL or 13–15 g/dL, using epoetin beta. After a 3-year follow-up, the need for dialysis was significantly greater in the patient with the higher hemoglobin target. However, there was also a significant improvement in the quality of life measure in the same.
The CHOIR trial , similarly conducted, looked at the frequency of cardiovascular events in 1432 CKD patients randomized to either a full or partial correction of hemoglobin, using epoetin alfa. The target hemoglobin was 13.5 g/dL in the full correction group as compared to 11.3 g/dL for the partial correction cohort. At 16 months, the trial was stopped prematurely when significantly more patients (N = 125) in the complete anemia correction group had more cardiovascular events as compared to the partial correction group (N = 97).
Finally, further confirmation of serious safety signals appeared in the TREAT trial [67]. This was an international effort to accurately and concisely answer the question whether normalizing hemoglobin with ESAs was harmful to CKD patients or beneficial. With the largest cohort to date, 4038 CKD patients with diabetes were randomized to either hemoglobin of 13 g/dL or placebo, using darbepoetin alfa. This placebo group received “rescue” darbepoetin when hemoglobin dropped below 9 g/dL. With a 29-month follow-up, there was no difference in the frequency of mortality or cardiovascular event rate in both groups. There was, however, a significantly higher rate of cerebral vascular events in the higher hemoglobin cohort as compared to the lower hemoglobin group. There was also a higher rate of venous thrombo-embolic events (deep vein thrombosis and pulmonary emboli) in the higher hemoglobin group and a trend toward higher mortality in patients with a history of malignancy. This trial ended any debate as to whether higher hemoglobin goal (>11 g/dL) achieved with ESAs was safer than rescue ESA use when hemoglobin dropped below 9 g/dL.
ESA Side Effects
One of the most commonly encountered side effects of ESA is hypertension. An increase of over 10 mmHg in diastolic blood pressure was encountered in as much as 35% of the patients enrolled in the original phase III trial of erythropoietin. Pooled data suggest that hypertension develops in about 20–30% of patients as a consequence of erythropoietin use [68]. There are many mechanisms by which ESAs can elevate blood pressure. These mechanisms include: the direct effect increasing hematocrit, changes in production or sensitivity to endogenous vasopressors, alterations in vascular smooth-muscle ionic milieu, dysregulation of production or responsiveness to endogenous vasodilatory factors, a direct vasopressor action of EPO, and finally arterial remodeling through stimulation of vascular cell growth [69]. The dominant mechanism seems to be an EPO-induced atrial hypertension with a dose-dependent increase in hypertension rate with the administration of EPO [70, 71].
Another commonly encountered adverse effect related to the use of ESAs is thrombosis. The etiology is mostly due to increasing viscosity brought on by increased hematocrit but also through a hematocrit-independent induction of platelet activation [72]. In dialysis patients, this translates into higher rates of cerebral vascular events, myocardial infarction, and graft thrombosis [67, 73]. Broadly, the rate of thrombosis was found to be approximately six times more frequent in patients receiving rHuEPO than those not receiving this therapy [74].
An additional untoward effect of ESA agents is the impact on vascular endothelium. Erythropoietin is associated with a direct vasoconstrictive effect on both the renal and mesenteric blood vessels. Additionally, stimulation of the V2 receptors can lead to an enhanced cell proliferation, and induction of proto-oncogenes [75, 76].
Dosing and Administration
Erythropoiesis-stimulating agents can be administered intravenously or subcutaneously, with equal efficacy. Most CKD patients receive the subcutaneous route due to ease of administration and a slightly longer half-life.
Current FDA regulations recommend ESA use only as a rescue treatment for hemoglobin <9 g/dL, predominantly in order to avoid complications related to transfusion and help alleviate anemia-induced clinical symptoms. Due to the substantial body of literature demonstrating significant morbidity and mortality in using these agents at higher hemoglobin targets (>11 g/dL) and an abundance of side effects.
The KDIGO anemia guidelines released in 2012 recommend tailoring treatment with ESAs for each patient by balancing the potential benefit of reducing transfusions and anemia-related clinical symptoms against the potential risk associated with ESA use (e.g., cerebral and cardiovascular events, vascular access loss, hypertension) [77]. Those guidelines refer to a large body of literature with a moderate quality of evidence suggesting that a modest hemoglobin target between 9 and 11 g/dL avoids the risk of repeat blood transfusion and poor quality of life while minimizing the reported risks of higher hemoglobin.
For all CKD patients with anemia requiring iron supplementation, a goal of transferrin saturation (TSAT) no greater than 30% and ferritin level no greater than 500 ng/mL should be achieved. Although most patients with >100 ng/mL (or TSAT >20%) will have adequate bone marrow stores, supplementing those patients may result in either improved hemoglobin level or decrease in ESA dose. There is evidence to suggest that patients with ferritin level as high as 1200 ng/mL can still benefit from IV Iron, but the KDIGO guidelines do not recommend it [78]. They also give a non-graded recommendation to avoid giving IV iron to patients with active infections.
Newer Agents
Much has been done to explore novel therapies that will correct anemia and avoid cardiovascular, cerebrovascular, and malignant side effects experienced with the use of ESAs. Several agents are in the process of evaluation which include hypoxia-inducible factors stabilizers, hepcidin inhibitors, and GATA-2 inhibitors.
Hypoxia-inducible factors (HIF ) are transcription factors that upregulate the expression of the erythropoietin gene. Under normal conditions, propyl hydroxylase (PHD) uses oxygen as a cofactor to hydroxylate HIF-α. This enables Von Hippel Lindau (VHL) tumor suppressor protein to target HIF-α and deliver it for proteasomal degradation. HIF propyl hydroxylase inhibitors prevent HIF from being hydroxylated and ultimately enzymatically degraded, which enables HIF to travel to the nucleus and induce transcription of the EPO gene (Fig. 6.1). In July 2015, the results of phase 2 trials with Roxadustat™, an oral HIF propyl hydroxylase inhibitor, were published. Ninety-six patients were compared to placebo; at 1.5 and 2 mg/kg dosing, Roxadustat™ induced a transient elevation in erythropoietin and reduced hepcidin expression, translating into a dose-dependent hemoglobin rise. The side-effect profile was similar to placebo [79]. One potential disadvantage of this therapy is in the induction of a multitude of other hypoxia-induced genes, such as those of vascular endothelial growth factor which may potentially lead to tumor growth and worsening diabetic proliferative retinopathy, although to date no evidence has been of this [80, 81].


Fig. 6.1
Mechanism of action of HIF stabilizers (also referred to as PHD inhibitors). Inhibition of propyl hydroxylase leads to stable hypoxia-inducible factors which can ultimately travel to the nucleus and induce epoetin production. PHD propyl hydroxylase, HIF hypoxia-inducible factor, VHL Von Hippel Lindau. (Reprinted from Kim SY, Yang EG. Recent advances in developing inhibitors for hypoxia-inducible factor prolyl hydroxylases and their therapeutic implications. Molecules. 2015;20(11):20,551–20,568. With permission from MDPI AG (Basel, Switzerland). Under the Creative Commons License: https://creativecommons.org/licenses/by/4.0/
Additionally, hepcidin is the major regulator of iron storage in the setting of active inflammation. Interleukin-6 induces its (mostly) hepatic production. Hepcidin regulates the amount of iron absorption by the duodenal enterocytes [82]. It also controls iron release from these and other cells including hepatocytes and macrophages in the reticuloendothelial system [83]. Hepcidin binds to ferroportin (a transmembrane iron exporter) and internalizes it, thereby preventing the iron release from these cells [84]. While still in early experimental phase, hepcidin antagonism as an anemia treatment has been researched. Monoclonal antibodies against hepcidin as well as direct mRNA-based antagonists of hepcidin have been developed [85]. Concerns about this modality over potential infectious risk are appropriately placed since hepcidin has an innate antimicrobial effect.
The GATA (guanine-adenine-thymine-adenine ) transcription factors are a family of transcription factors characterized by their ability to inhibit EPO gene expression through binding the GATA DNA sequence. Of particular importance is the GATA-2 transcription factor that has experimentally been inhibited by two experimental inhibitors K-7174 and K11706, leading to improved anemia markers in experimental animal models [86, 87]. Compound K-7174 s mechanism of action has been linked to inhibition of hepcidin [88].
With multiple experimental and novel therapeutic agents, only the HIF stabilizer has made a significant leap into applicable medicine with the phase 4 trials currently underway.
Regulatory Impact on Anemia Management
While this chapter stresses the importance of evidence in driving decisions to manage CKD patients with anemia, the impact of funding and policy on actual medical practice cannot be ignored. It is important to explore how policy has ultimately had such an invasive but integral regulatory impact on medical practice. In 1967, the Gottschalk report argued the need for Medicare reimbursement for dialysis and transplant as established treatments and not. It was not until October 30th of 1972 that President Richard Nixon signed an amendment to social security payment extending Medicare coverage to those with chronic kidney disease. Finally, these patients could put their concerns about the financial burdens of their treatment behind them. With the advent of epoetin, in 1989, there was concern that, as a separately billable pharmaceutical, that there may a conflict between the financial benefit to maximize its use and known clinical benefit(s). In 1994, Medicare expenditure for epoetin therapy alone exceeded 700 million dollars [89]. However, the increasing expansion in ESA dosing did not translate into higher hemoglobin levels, and in 1993, concerned about the increase in financial expenditure, the Centers for Medicare and Medicaid Services (CMS) initiated the National Anemia Cooperative Project [90]. Published in 1996, this was an expert opinion-based anemia treatment algorithm that emphasized target hematocrit >30% with emphasis on iron replacement [91, 92]. In 1997, the National Kidney Foundation (NKF) published the first evidence-based guideline—Dialysis Outcomes Quality Initiative (DOQI) anemia guideline.
In 2007, following the publication of both the CHOIR and CREATE trials, the food and drug administration (FDA) issued a black box warning for ESAs further limiting its use and consequently cost. The FDA advised health care professionals to use the lowest dose that will gradually increase the hemoglobin concentration to the lowest level sufficient to avoid the need for red blood cell transfusions. The FDA also warns that ESAs increased the risk for death and for serious cardiovascular events when administered to target hemoglobin of greater than 12 g/dL.
Considering regulatory impact and the evidence against driving higher hemoglobin levels with erythropoietin, the current trend in the management of CKD patients with anemia is essentially geared at minimizing transfusions with target hemoglobin above 9 g/dL. The ultimate goal is always to tailor treatment for each patient. This translates into minimizing the impact of low hemoglobin on the patient’s quality of life (i.e., improving dyspnea, fatigue, sleep disturbances, and cognitive decline) while concomitantly, avoiding the use of exaggerated or supratherapeutic doses of ESAs in order to achieve an idealized hemoglobin level.
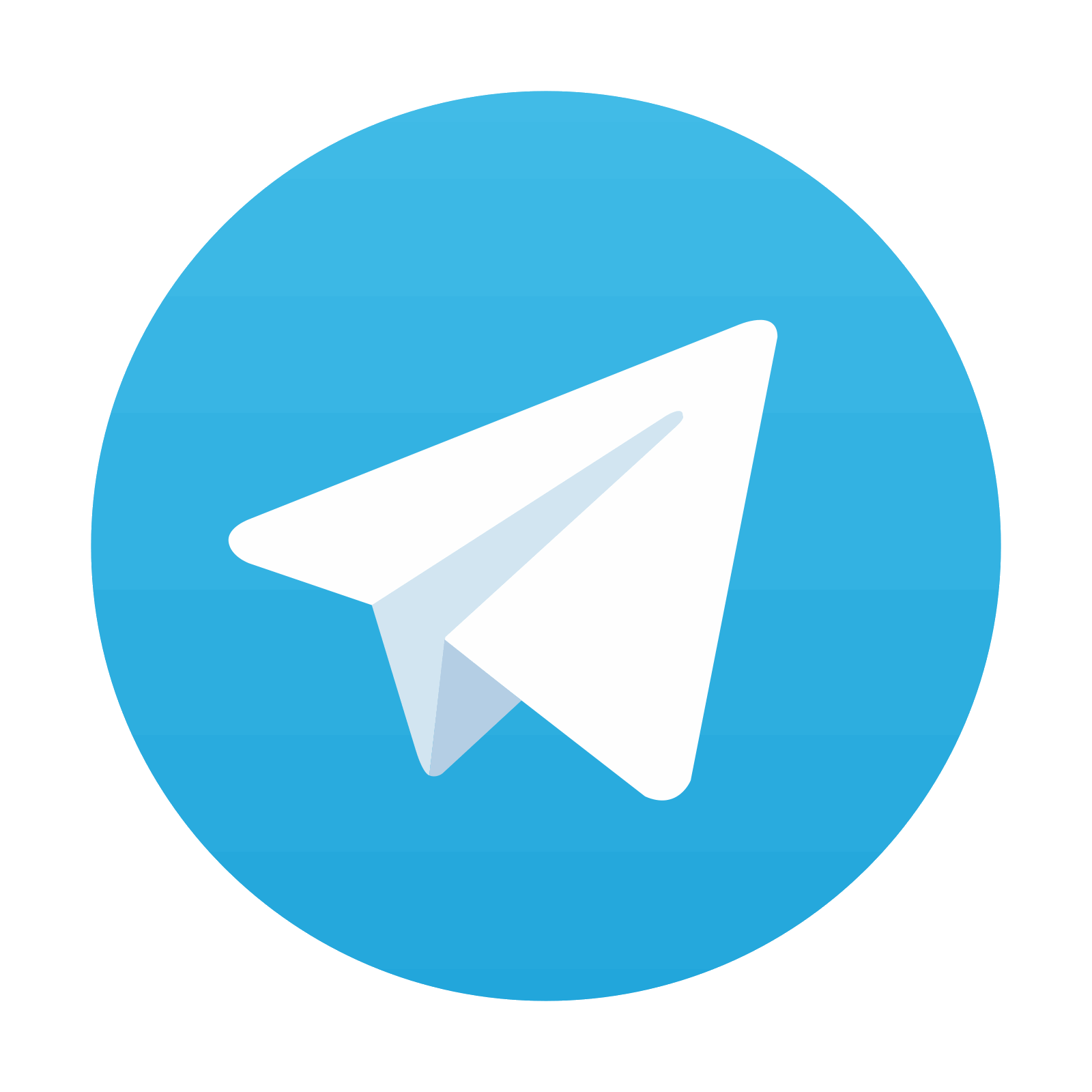
Stay updated, free articles. Join our Telegram channel
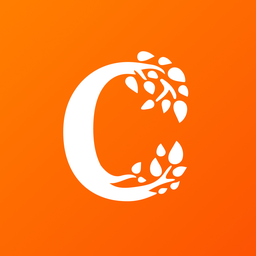
Full access? Get Clinical Tree
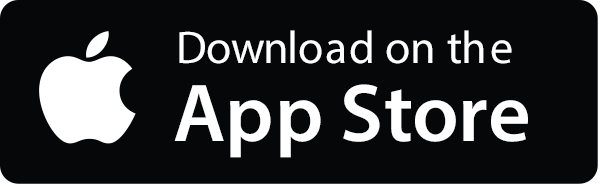
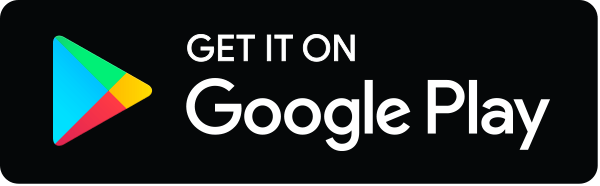