div class=”ChapterContextInformation”>
8. Physiology and Pharmacology of the Prostate
Keywords
ProstateUrinary physiologyProstate-specific antigenSympathetic nervous systemAdrenergic alpha blockersNitric oxidePhosphodiesterase 5 inhibitors5 Alpha reductase inhibitorsAndrogen receptorIntroduction
The prostate is located in the lower pelvis and positioned between the bladder neck and pelvic floor. The prostate is a tubulo-alveolar gland, part of both the reproductive and urinary system, and universally present in mammals. The urethra of humans runs in cranio-caudal direction through the prostate (i.e. prostatic urethra) and the ejaculatory ducts run on both sides from dorso-cranial to ventro-caudal direction towards the prostatic urethra. The prostate grows and matures during puberty under the influence of testosterone from a small gland of 2–5 cm3 in childhood to a gland volume of approximately 20–25 cm3 in early adolescence [1]. Approximately 15–30 ejaculatory ducts disembogue at the verumontanum which is located on the dorsal side halfway through the prostatic urethra. Based on the position of the verumontanum, the prostatic urethra can be divided into a proximal and distal part which has in the young healthy adult a length of approx. 1 cm each. Starting at the verumontanum and ventrally directed, the proximal prostatic urethra has an angle of approximately 35° [2, 3].
- 1.
Anterior zone: this area does not have glands and only contains muscular and fibrotic cells. The aglandular prostate forms the ventral surface of the prostate and is inseparable fused with the glandular prostate. The function of this area remains unknown but strong muscular components suggest contracting abilities.
- 2.
Central zone: constitutes approximately 25% of the glandular prostate in young, healthy adults, has a conical shape with the tip of the cone directed towards the verumontanum and the base towards the seminal vesicles. Glandular tubes run parallel to the intra-prostatic ejaculatory duct and disembogue in the center of the verumontanum. The glands are oriented in a coronal plane and contain wide chambers. The epithelium of the glands has multiple rows.
- 3.
Peripheral zone: constitutes approximately 70% of the glandular prostate in young, healthy adults and surrounds the central zone from the base to the apex. The ducts radiate laterally from the urethra and disembogue lateral and distal of the verumontanum. The glands have a uniform configuration, only a few branches and a round lumen. The epithelial cells are long and positioned in a single row and have a uniform shape as well as a light cytoplasm.
- 4.
Transition zone: constitutes approximately 5% of the glandular prostate in young, healthy adults and is positioned on the dorsal side of the proximal prostatic urethra. The transition zone only has a few ducts which run parallel to the urethral axis. The glands are straight and the epithelial cells have a similar shape compared to those of the peripheral zone; however, the interstitial tissue is irregular.

TUR specimen of a patient with benign prostatic hyperplasia demonstrating the different tissue components of the transition zone (hematoxylin eosin staining, enlargement 150-fold). Prostatic glands with single layer epithelial cells appear blue and the lumen white (without secretion, washed out during fixation). The glands are separated by interstitial tissue with a large amount of smooth muscle cells (red). A glandular duct with single layer epithelial cells runs in vertical direction through the tissue. Elastic and collagen fibers as well as lymphatic vessels and nerves are not stained and remain invisible on this image (courtesy of Dr. S. Afram, Pathological Institute Gronau, Germany)

Schematic illustration of the gross anatomy of the prostate, imaged 45 degrees from the left (zonal anatomy according to McNeal [4, 5]). The anterior fibromuscular stroma (AFS) is positioned ventrally. The central zone is located dorsally of the urethra and is surrounded by the peripheral zone. The transition zone is positioned at the proximal urethra (proximal of the verumontanum, i.e. the location where the ejaculatory ducts (ED) disembogue into the urethra). The seminal vesicles (SV) are located dorso-laterally of the prostate on both sides (modified according to Roehrborn et al. [6])
The different morphology of the prostatic glands and different appearances of epithelial cells in different zones indicate different functions within the glandular prostate [10, 11]; however, it remains largely unknown which zones are responsible for particular functions or secretions in the context of ejaculation and reproduction.
Different diseases develop in different zones. Prostate cancer and prostatitis begin in the peripheral zone [12], whereas benign prostatic hyperplasia (BPH) originates in the transition zone [13]. However, the cause of BPH remains largely unknown. The prevalence of BPH increases with aging [1] and may result in enlargement of the transition zone and secondarily in enlargement of the entire prostate (i.e. benign prostatic enlargement, BPE), elongation of the proximal prostatic urethra (>1 cm), increase of the ventrally directed angle of the proximal prostatic urethra (>35°), increased urethral resistance of urine flow (i.e. benign prostatic obstruction, BPO) and lower urinary tract symptoms (LUTS) [2, 3, 14, 15].
Physiology of the Prostate
The prostate is—next to the seminal vesicales and the bulbourethral glands (Cowper)—part of the male accessory sex glands and produces seminal fluids. Smooth muscle cells of the prostate contract during orgasm in order to expel ejaculate through the urethra [16]. Epithelial cells of the prostatic glands produce a secretion that empties during ejaculation through the glandular ducts into the prostatic urethra where they mix with semen from the testes and epididymes as well as with secretions from the seminal vesicales [16].
Prostatic secretion is an important component of the ejaculate and constitutes approximately 25–30% of the ejaculate volume (~0.5 mL). The prostatic secretions are a milky white mixture of simple sugars (e.g. fructose and glucose), proteins, minerals and alkaline chemicals which protect and nourish sperm. The sugars secreted by the prostate function as nutrition for the spermatozoa while they pass into the female body to fertilize eggs [16]. Protein content is less than 1% of the total prostatic secretion and includes proteolytic enzymes, prostate-specific antigen (PSA), prostatic acid phosphatase and β-micro-seminoprotein [17]. PSA, a glycoprotein and member of the kallikrein-related peptidase family produced and secreted in prostatic epithelial cells, and other enzymes break down seminal proteins to free spermatozoa from the viscous semen, thereby making semen thinner and allowing sperm cells to swim freely. Additionally, PSA can also dissolve cervical mucus in order to give sperm cells free passage to the uterus to fertilize eggs. The secretions also contain zinc with a concentration 500–1000 times the concentration in blood [16]. The alkaline chemicals in prostatic secretions (~pH 7.8) neutralize acidic vaginal secretions to promote the survival and prolong the lifespan of spermatozoa in the female body, thereby increasing their chance of successfully fertilizing an egg [16].
The prostate needs hormones (androgens) to function properly. The predominant male sex hormone is testosterone which is mainly produced by the Leydig cells of the testes, to a lesser amount also in the adrenal glands. Testosterone is metabolized into the active hormone dihydrotestosterone (DHT) inside prostatic epithelial cells by the enzyme 5α-reductase (see Section “Androgens”).
Pharmacology of the Prostate
The prostate contains several tissue types (especially smooth muscle cells and epithelium) which are rich of receptors and enzymes. Targeting these receptors/enzymes helps treating LUTS/BPH or PCa. LUTS/BPH is the fourth most common disease in men aged ≥50 years and prostate cancer is the most common malignancy in males [18]. Therefore, the following targets and the selective manipulation of these became most important for all urologists and also general practitioners.
Adrenergic Receptors (Adrenoceptors)
The Sympathetic Nerve System

Schematic illustration of the sympathetic nervous system (left) and enlargement of the neuromuscular connection (right). After depolarization of the postganglionic neuron, noradrenaline (NA) is released into the synaptic gap. NA molecules bind to α- and β-adrenoceptors on the postsynaptic membrane where they initiate effects (e.g. smooth muscle cell contraction). NA release is terminated via a negative feedback mechanism mediated by α2-andrenoceptors on the presynaptic membrane (modified according to Oelke et al. [21])
α1-Adrenergic Receptors
The human prostate predominantly contains α1-adrenoceptors, to a lesser amount also α2-adrenoceptors [26–29]. α1-Adrenoceptors are located on smooth muscle cells (proportion of α1:α2 = 4:1), whereas α2-adrenoceptors are mainly located in the epithelium and blood vessels [26, 28, 30]. Functional studies clarified that smooth muscle cell contraction in the prostate is mediated by α1-adrenoceptors [31–35]. Hyperplastic prostatic tissue (BPH) has more smooth muscle cells (absolute and in percentage) and carries more α-adrenoceptors than normal prostate tissue [34, 36, 37]. However, the content of α1-adrenoceptors in the prostate of BPH-patients with LUTS is identical to BPH-patients without LUTS; therefore α1-adrenoceptors do not seem to be the origin of LUTS [32].
Pharmacologically interesting for the treatment of LUTS/BPH are especially the α1-adrenoceptors of which three subtypes have been identified, the α1A-, α1B– and α1D-adrenoceptors [38–40]. Historically, the α1C-adrenoceptor was also described but turned out to be identical with the α1A-subtype; therefore, the exact specification of this α1-adrenoceptor subtype was abandoned [41, 42]. Approximately 70% of α1-adrenoceptors in the prostate are α1A-subtypes [43] which are predominantly located on smooth muscle cells [41]. The α1A-adrenoceptors increase numerically and the proportion of α1A:α1B:α1D adrenoceptor subtypes changes from 63:6:31 in normal prostatic tissue to 85:1:14 in BPH tissue [44]. Inhibition of the α1A-adrenoceptor subtype results in smooth muscle cell relaxation. The α1B-adrenoceptor is mainly located in blood vessels and inhibition of this adrenoceptor subtype is associated with vasodilation [45, 46]. The α1D-adrenoceptor is predominantly expressed in the bladder, peripheral ganglia and spinal cord; inhibition of this adrenoceptor subtype results in direct or indirect (peripheral ganglia, spinal cord) relaxation of the detrusor [47–49].
α1-Adrenergic Receptor Antagonists (α1-Blockers)
It was shown in patients with BPH that smooth muscle cells account for ~39% of the cellular volume of the prostate and ~51% of the stroma volume [50]. The aim of the treatment of LUTS/BPH is to relax smooth muscle cells of the prostate and bladder neck by inhibition of α1A-adrenoceptors which eventually decrease urethral resistance. Inhibition of the adrenergic innervation of the prostate can reduce the intra-prostatic urethral pressure by approx. 47% [51–53] and can improve the detrusor pressure at maximum urine flow (Pdet.Qmax) by approximately 11.4 cm H2O, maximum urine flow (Qmax) by 2.3 mL/s as well as bladder outlet obstruction index (BOOI = Pdet.Qmax − 2Qmax) by 14.2 cm H2O [54].

Commercially available α-adrenoceptors antagonists and their subtype selectivity. Only the α1-adrenoceptor antagonists in blue color are currently licensed for the treatment of “symptoms or signs of BPH” (modified according to Oelke et al. [21])
Key pharmacokinetic properties and standard doses of α1-blockers licensed in Europe for the treatment of “signs or symptoms of BPH” [62]
Drug | tmax (h) | t½ (h) | Recommended daily dose (mg) |
---|---|---|---|
Alfuzosin IR | 1.5 | 4–6 | 3 × 2.5 |
Alfuzosin SR | 3 | 8 | 2 × 5 |
Alfuzosin ER | 9 | 11 | 1 × 10 |
Doxazosin IR | 2–3 | 20 | 1 × 2–8 |
Doxazosin GITS | 8–12 | 20 | 1 × 4–8 |
Silodosin | 2.5 | 11–18 | 1 × 4–8 |
Tamsulosin MR | 6 | 10–13 | 1 × 0.4 |
Tamsulosin OCAS | 4–6 | 14–15 | 1 × 0.4 |
Terazosin | 1–2 | 8–14 | 1 × 5–10 |
Clinical Efficacy of α1-Blockers
Although LUTS and urine flow improvements take a few weeks to fully develop, statistically significant and clinically relevant differences compared to placebo were already documented within a few hours or days after first drug intake [67]. α1-Blockers show a similar efficacy, expressed as percent improvement in the International Prostate Symptom Score (IPPS) questionnaire, in patients with mild, moderate or severe LUTS [68]. RCTs demonstrated that α1-blockers reduce LUTS (both storage and voiding symptoms) by ~30–40% and increase Qmax by ~20–25% after the placebo run-in period [62]. In observational studies (without a placebo run-in period), IPSS improved by ~50% and Qmax by ~40%. Indirect comparisons and limited head-to-head comparisons between α1-blockers indicate that all α1-blockers have a similar efficacy when used in appropriate doses [69, 70]. However, a recent network meta-analysis suggested that some α1-blockers reduce LUTS to a greater extent than others [71]; mean IPSS reduction was −7.1 points for doxazosin and −6.8 points for terazosin, whereas mean IPSS reduction for silodosin, alfuzosin, tamsulosin and naftopidil was −5.8, −5.5, −5.5 and − 5.4 points, respectively. This preliminary data needs confirmation by independent analyses, especially considering the different doses and formulations of the individual α1-blockers.
α1-Blockers do not reduce prostate size and, additionally, prostate size does not affect α1-blocker efficacy in studies with follow-up period of ≤1 year [62, 72]. Qmax at baseline does not have relevant influence on LUTS reduction [73]. Long-term efficacy of α1-blockers was documented for a period of longer than 4 years [74]. Nevertheless, α1-blockers are not able to prevent acute urinary retention (AUR) in the long run, especially in patients with a prostate volume >40 cm3 (see Section “Androgens”) [74, 75].
Men with BPH can develop AUR due to increased bladder outlet resistance (BPO) and/or insufficient detrusor function (detrusor underactivity). The incidence rate is approximately 2.2 cases/1000 patient-years in asymptomatic and 18.3–35.9 cases/1000 patient-years in symptomatic men [76]. The use of α1-blockers in these patients allows approx. 60% of men to void spontaneously again compared to approx. 38% using placebo (successful trial without catheter, TWOC), which measures up to a 55% increase in the success rate [77].
For further reduction of LUTS, α1-blockers can be combined with drugs of other drugs classes, e.g. with phosphodiesterase type 5 inhibitors (PDE5i; see Section “Phosphodiesterases”) [78–80], 5α-reductase inhibitors (5ARIs; see Section “Androgens”) [75, 81], muscarinic receptor antagonists (antimuscarinics) [82, 83] or β3-receptor agonists [84].
Adverse Event Profile of α1-Blockers
Although alfuzosin, doxazosin and terazosin have a similar molecular structure (quinazoline-based derivatives) and do not selectively inhibit specific α1-adrenoceptor subtypes, the adverse event profile of alfuzosin is more similar to tamsulosin (non-arylamine sulfonamide derivative) than to doxazosin or terazosin. The mechanisms underlying such differential tolerability are not fully understood but may involve better penetration and/or tissue distribution by alfuzosin and tamsulosin [62]. Other factors, such as subtype selectivity and the pharmacokinetic profiles of certain formulations, may also contribute to the adverse event profile of individual drugs.
The most frequently reported adverse events of α1-blockers are asthenia, dizziness and (orthostatic) hypotension which can be explained by the inhibition of α1B-adrenoceptor subtypes in vessels, leading to vasodilation and blood pressure decrease [85]. At least some of the observed asthenia and dizziness can be attributed to blood pressure decrease. In particular, patients with cardiovascular co-morbidity and/or vasoactive co-medication appear to be prone to vasodilatation during α1-blocker treatment [86]. This includes anti-hypertensive drugs, such as diuretics, Ca2+-channel blockers, β-blockers, angiotensin-converting enzyme inhibitors and angiotensin receptor antagonists but also phosphodiesterase type 5 inhibitors (PDE5i) prescribed for erectile dysfunction or male LUTS. Vasodilatation is more pronounced with immediate-release doxazosin and terazosin but less common for alfuzosin or tamsulosin (odds ratio for vascular-related adverse events 3.3, 3.7, 1.7 and 1.4, respectively; the latter two not reaching statistical significance [87]). In contrast, blood pressure decrease or prevalence of orthostatic hypotension with the α1A-selective blocker silodosin is comparable with placebo [85, 88] and, therefore, this drug can safely be used with anti-hypertensive co-medication [89].
The intraoperative floppy iris syndrome (IFIS) was only discovered in 2005 in the context of cataract surgery [90]. IFIS consists of a typical triad of the following intraoperative characteristics: (1) a flaccid iris stroma leading to fluttering and billowing of the iris, (2) a tendency for the floppy iris stroma to prolapse through the surgical incision and (3) a progressive pupil constriction despite standard perioperative pharmacologic measures for prevention. Most patients, however, manifest an incomplete form of this triad displaying only one or two signs [85]. The basis of IFIS is thought to be antagonism of the α1-adrenoceptor subtype located in the iris dilator muscle. The incidence varies between 30 and 88% for tamsulosin, 15 and 70% for alfuzosin, and 2 and 45% for doxazosin users. In a meta-analysis, alfuzosin, doxazosin, tamsulosin and terazosin had an increased risk for IFIS [91]. The odds-ratio for IFIS was 393 for tamsulosin, 9.7 for alfuzosin, 6.4 for doxazosin and 5.5 for terazosin. Silodosin has yet not been associated with IFIS [85]. α1-Blocker treatment should not be initiated prior to scheduled cataract surgery, and the ophthalmologist should be informed about previous or current α1-blocker use [85]. Important to mention is that the occurrence of IFIS may complicate cataract surgery and make it technically more challenging but no reports about increased health risks of these patients have been published.
A systematic review concluded that α1-blockers do not adversely affect libido, sometimes have a small beneficial effect on erectile function (e.g. doxazosin) but can cause abnormal ejaculation [92]. Originally, abnormal ejaculation was thought to be retrograde but more recent data demonstrate that it is due to (relative) anejaculation (i.e. reduction or absence of seminal fluids during ejaculation). Abnormal ejaculation seems to be caused by α1A-adrenoceptor subtype selective inhibition of smooth muscle cells in the prostate, seminal vesicles, ejaculatory ducts and spermatic cords and is more frequently observed with silodosin and tamsulosin than with other α1-blockers. LUTS reduction was shown to be higher in men with abnormal ejaculation compared to men without [93]. The apparently greater risk for abnormal ejaculation with tamsulosin is intriguing as even the more α1A-selective drug silodosin carries an even greater risk [94, 95]. However, all α1-blockers are dosed to block α1A-adrenoceptors effectively; therefore, the mechanism underlying abnormal ejaculation still remains to be elucidated.
A dose-dependent increased risk of dementia for tamsulosin was only recently described (2018) in a cohort analysis of more than 250,000 US-American patients after a follow-up of 20 months (hazard ratio 1.11–1.20; 38.8 cases/1000 patient years) [96]. In contrast, an increased risk for dementia was not seen for other α1-blockers (alfuzosin, doxazosin or terazosin) or 5ARIs (finasteride or dutasteride). Another recently published cohort study (2019) with approx. 60,000 Korean patients with a follow-up of 52 months could not confirm the results seen in the North American study [97]. However, the daily standard dose for tamsulosin in Asian patients is only 0.2 mg, and differences in pharmacokinetics, metabolism and genetic/ethnicity may have contributed to these controversial results. Therefore, the association between α1-blocker use and dementia still needs to be clarified in future studies.
Phosphodiesterases
The NO/NOS: Phosphodiesterase System

Cellular responses (e.g. smooth muscle cell relaxation) after activation of protein kinase A (PKA) by cyclic adenosine monophosphate (cAMP-pathway, left) or protein kinase G (PKG) by cyclic guanosine monophosphate G (cGMP-pathway, right). The effect of cAMP is terminated by phosphodiesterase type 4 (PDE 4) and the effect of cGMP is terminated by phosphodiesterase type 5 (PDE 5). PDE 4 inhibitors (PDE 4i; e.g. rolipram, Ro 20-1724 or RP 73401) block the degradation and thus inactivation of cAMP. PDE 5 inhibitors (PDE 5i; e.g. avanafil, sildenafil, tadalafil, udenafil or vardenafil) block the degradation and thus inactivation of cGMP, which results in accumulation of cyclic monophosphates and prolonged cellular responses (modified according to Ghofrani et al. [100] and Lincoln et al. [101]). AMP adenosine monophosphate, cAMP cyclic adenosine monophosphate, ATP adenosine triphosphate, GMP guanosine monophosphate, cGMP cyclic guanosine monophosphate, GTP guanosine triphosphate, NO nitric oxide, NOS nitrite oxide synthases, PDE phosphodiesterase, PDEi phosphodiesterase inhibitor, PKA protein kinase A, PKG protein kinase G
PDEs are classified according to their preferences for cAMP or/and cGMP, kinetic parameters of cyclic nucleotide hydrolysis, sensitivity to the inhibition by various compounds, allosteric regulation by other molecules, and chromatographic behavior on anion exchange columns [99]. In total, 11 families of PDE isoenzymes have been identified in humans (PDEs 1–11). Some of these isoenzyme families have more than one gene and some genes are alternatively spliced so that more than 50 isoenzymes or variants have been identified. Some PDE genes are also variably expressed in different tissues [104]; therefore, the distribution and functional significance of PDE isoenzymes vary in different organs and, consequently, isoenzyme-selective inhibitors have the potential to exert specific effects on target tissues. In the lower urinary tract, the cAMP- and/or cGMP-specific PDE isoenzymes PDE 1, PDE 2, PDE 4, PDE 5 and PDEs 7–10 have been identified of which PDE4 (cAMP-specific PDE) and PDE5 (cGMP-specific PDE) are the predominant ones in the prostate, bladder and urethra [105]. Immunohistochemistry showed that PDEs 4 and 5 are mainly located in stromal and glandular tissues of the transition zone. A clinical study with 30 men with moderate to severe LUTS awaiting transurethral resection of the prostate (TURP) were randomly divided to receive 20 mg tadalafil or 200 mg udenafil 1 h prior to TURP or TURP without the preceding administration of a PDE5i. The concentrations of the PDE5i and cGMP were measured in the plasma and resected prostate specimen and, afterwards, the prostate tissue/plasma ratio was calculated. The authors showed that (1) the PDE5i was predominantly distributed in the prostate (transition zone) and (2) tadalafil and udenafil significantly increased cGMP levels in both the plasma and prostate tissue.
Organ bath studies demonstrated that, after α1-adrenergic stimulation of smooth muscle cells with noradrenaline, the tension of prostate tissue strips was dose-dependently reversed by PDE4 inhibitors (e.g. rolipram, Ro 20-1724 and RP 73401) and PDE5 inhibitors (e.g. sildenafil, tadalafil and vardenafil) and accompanied by an increase of cAMP or cGMP levels in the tissue [105–107]. These results indicate that cAMP and cGMP as well as PDEs 4 and 5 are involved in relaxation of prostatic smooth muscle cells.
The NO/NOS- and cAMP/cGMP-systems can also relax smooth muscle cells outside the lower urinary tract, for example in arteries, leading to vasodilatation and increased blood circulation. This is an important hint because it has been postulated that hyperplasia of myocytes, fibrocytes and epithelial cells—as seen in BPH tissue—may be induced by hypoxia resulting from an age-related impairment of blood flow in the small pelvis. Consequently, a key role of urogenital ageing and subsequent alterations in the blood supply of the prostate has been suggested for the development of BPH [108]. Transrectal contrast-enhanced color Doppler ultrasound studies demonstrated that perfusion of the transition zone of the prostate was significantly lower and mean flow resistance index significantly higher in men with BPH than in healthy controls [109]. Thus, it seems likely that regular administration of PDE5i may, to a certain degree, overcomes ischemia due to vascular damage and increase local blood flow by relaxation of smooth muscle cells in pelvic arteries [110].
Phosphodiesterase Type 5 Inhibitors (PDE5i)
PDE5i have a similar structure to cGMP and inhibit the breakdown of NO-derived cGMP molecules by competitively binding the catalytic site of PDE5, thereby causing accumulation of cGMP in the cell (cytoplasm) for continuous activation of the NO/cGMP system and, hence, prolonged (prostatic) muscle cell relaxation (Fig. 8.5).
Phosphodiesterase type 5 inhibitors (PDE5i) licensed in Europe for the treatment of “erectile dysfunction” and/or “signs or symptoms of BPH”; key pharmacokinetic properties and doses used in clinical trials [62, 112]
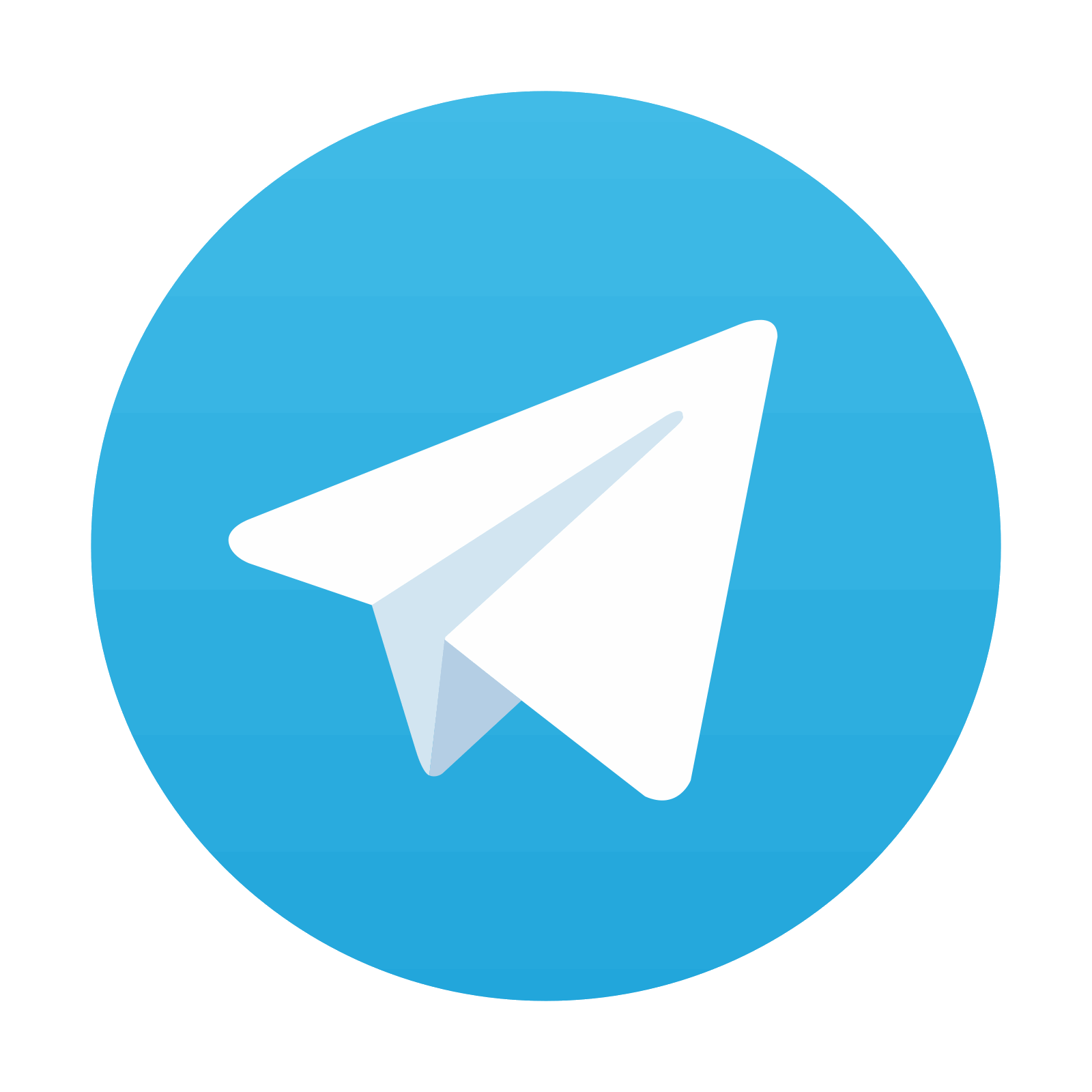
Stay updated, free articles. Join our Telegram channel
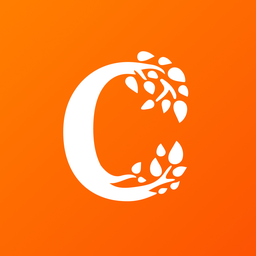
Full access? Get Clinical Tree
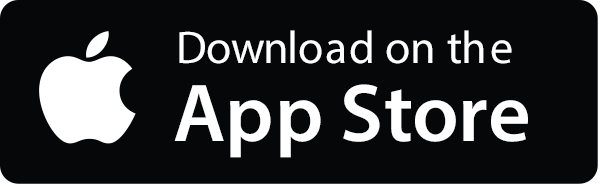
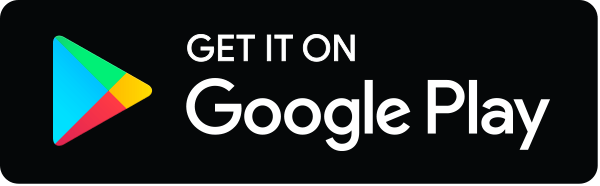
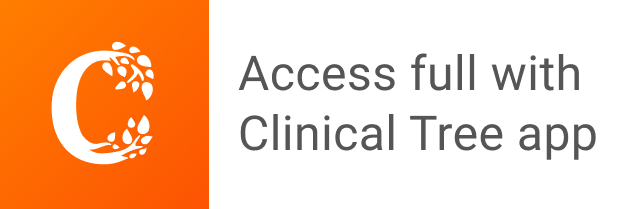