Abstract
Large quantities of fluid are transported across epithelial barriers in the gastrointestinal (GI) tract for secretion of saliva, gastric juice, bile and pancreatic fluid, and for fluid absorption in the intestine. The molecular pathways for water transport in the GI tract have been identified relatively recently and remain an evolving and in some cases controversial subject. As in other organ systems, the general paradigm in the GI tract is that water movement occurs secondary to osmotic driving forces created by active salt transport and to hydrostatic pressure differences. This chapter describes current concepts in fluid transporting mechanisms, from the original standing-gradient model to newer speculative models involving water pumps, electroosmosis, and osmosensing feedback. This chapter also describes potential roles for aquaporin (AQP) water channels in GI fluid transport. The AQPs are a family of small, integral membrane proteins that transport water and in some cases water and small solutes such as glycerol and hydrogen peroxide. AQPs are expressed widely in cell plasma membranes in epithelial, endothelial, and other cell types in the GI tract and elsewhere, including in salivary gland, liver, pancreas, gallbladder, and intestine. There is compelling evidence for the physiological importance of some AQPs in some tissues based on studies in humans with AQP deficiency/mutations, and phenotype analysis of transgenic mouse models of AQP deletion. In the GI tract, there is good evidence for the involvement of AQPs in salivary gland secretion and intestinal epithelial repair, though little evidence for other proposed functions such as intestinal fluid transport, and bile and pancreatic fluid secretion. AQPs are strongly expressed in GI tumors, though their role in tumor growth and spread remains unproven. Finally, the potential utility of AQP-targeted therapeutics, such as gene delivery and small molecule AQP-modulating drugs, is discussed.
Keywords
Fluid transport, Absorption, Secretion, Aquaporin, Intestine, Colon, Saliva, Bile, Pancreas, Osmotic
55.1
Introduction
Large quantities of fluid are transported across epithelial barriers in the gastrointestinal (GI) tract for secretion of saliva, gastric juice, bile and pancreatic fluid, and for fluid absorption in the intestine. The quantity of fluid transported in the GI tract is second only to kidney, where ~ 180 L of fluid per day are filtered by the glomerulus in humans and processed by various nephron segments. In the human GI tract, the salivary glands produce ~ 1.5 L of fluid per day, the stomach secretes 2.5 L of gastric juice, the liver produces 0.5 L of bile, the pancreas produces 1.5 L of enzyme and bicarbonate-rich fluid, the small intestine absorbs 7.5 L of fluid, and the colon absorbs 1.3 L of fluid against significant osmotic gradients ( Fig. 55.1 ). The fluids transported across epithelial and endothelial barriers contain salts (~ 150 mM) and water (~ 55,000 mM). As discussed elsewhere in this volume, there is a considerable body of data on the molecular identities of the major salt transporters in epithelial cells of the GI tract and their role in transcellular ion transport. The molecular pathways for water transport in the GI tract have been identified relatively recently and remain an evolving and in some cases controversial subject. As in other organ systems, the general paradigm in the GI tract is that water movement occurs secondary to osmotic driving forces created by active salt transport and to hydrostatic pressure differences. Based on a substantial body of evidence in the kidney and other epithelia carrying out active near-isosmolar fluid secretion or absorption, greater cell membrane water permeability produces greater net fluid movement. A description of current concepts in fluid transporting mechanisms is described in the section, “Epithelial fluid transporting mechanisms” to follow.

Aquaporin (AQP) water channels provide the molecular pathway for cell membrane water transport in many cell types. The AQPs are a family of small, integral membrane proteins that transport water and in some cases water and small solutes such as glycerol and hydrogen peroxide (H 2 O 2 ). AQPs are expressed widely in cell plasma membranes in epithelial, endothelial, and other cell types in the GI tract and elsewhere. As described in this chapter, there is compelling evidence for physiological importance of some AQPs in some tissues based on studies in humans with AQP deficiency/mutations, and phenotype analysis of transgenic mouse models of AQP deletion. However, the role of AQPs in the GI tract remains to some extent uncertain despite a considerable body of data on the expression pattern, cellular processing and regulation of AQPs in various GI cell types. The available data are reviewed in this chapter, and major unresolved questions are identified.
55.2
Epithelial Fluid Transporting Mechanisms
55.2.1
Introduction
The mechanism of how water is absorbed and secreted across epithelia has puzzled physiologists for decades. Although large volumes of water cross the various epithelia of the GI tract as diagrammed in Fig. 55.1 , there remains a lack of consensus about the mechanisms by which water is transported across epithelia both generally and in specific regions of the GI tract. However, the general paradigm of water transport following active solute movement has remained the basis of most models of fluid transport.
The original observation that fluid can be transported in the apparent absence of an osmotic gradient was made in a series of elegant studies by a few pioneering physiologists in the late 19th century. It was demonstrated that fluid could be transported across an epithelial sheet such as rabbit ileum in the absence of an external osmotic pressure difference, and that this transport occurred only as long as the tissue remained viable, implying that “active” metabolism within the tissue is required. In the middle of the 20th century, Ussing demonstrated that sodium is actively transported across epithelia, providing the basis for a model of fluid transport. Curran was the first to show that water transport was linearly related to Na + transport, and he and McIntosh thereafter proposed a “three-compartment model” ( Fig. 55.2 A ) of fluid transport that accounted for the ability of epithelia to transport water in the absence or against (“uphill”) an osmotic gradient. The model requires active solute transport from compartments I to II across membrane barrier A. Compartment II is then hypertonic to compartment I. If the reflection coefficient for the actively transported solute on membrane A is greater than that on membrane B, fluid will be transferred from compartments I to III in the absence of an osmotic pressure difference between compartments I and III.

55.2.2
“Standing Gradient” Model
Following the anatomical observations of Diamond and Whitlock and Wheeler, Diamond and Bossert extended the Curran/McIntosh model by delineating the biological structures responsible for the different compartments and membranes within the epithelium, resulting in the “standing- gradient” model ( Fig. 55.2 B). The model suggested that the lateral intercellular space (LIS) between adjacent epithelial cells was the central hypertonic compartment in the Curran-McIntosh model. The basic feature of the model as illustrated in Fig. 55.2 B is that the driving solute (Na + in most cases) is actively transported from the lumen into the LIS, resulting in a steady-state (standing) osmotic gradient decreasing from the tight junction to the basolateral membrane. The osmotic gradient drives water flux through the cells into the LIS and across the basolateral membrane, resulting in an isosmolar or near-isosmolar absorbate.
As originally conceived, the model predicted a number of important characteristics for an epithelium to absorb water in the absence of an osmotic gradient between the luminal and basolateral solutions, which include a water impermeable tight junction between cells, relatively low water permeability for membranes facing the LIS, and clustering of Na + /K + ATPase pumps near the tight junction. However, subsequent experimental data indicated that many of the original requirements of the model were incorrect, leading to revision of the original model and the development of alternative models. Hydraulic conductivity (Lp) measurements of various intestinal membranes have generally shown relatively high water permeabilities for both apical and basolateral membranes. This observation, together with a series of optical studies of water and solute dynamics in the LIS, led to a revised model in which the osmotic gradient localized in the LIS is predicted to be so small (< 10 mOsm) as to be essentially unmeasurable. Another tenet of the revised model is that there is a much greater magnitude of water transport through the cell than through tight junctions.
The validity of the revised “standing-gradient” model has remained uncertain, with an increasing number of theoretical inconsistencies and problems related to the difficulty in measuring many of the key parameters in physiologically relevant epithelial cells in vitro or in vivo. Reported water permeabilities of apical and basolateral membranes have differed over orders of magnitude, introducing considerable uncertainty about osmotic gradients required to drive fluid absorption. Also, the methodologies used to measure membrane water permeability have been criticized as has the physiological relevance of the model systems. The high water permeabilities measured by Spring and coworkers in Necturus gallbladder and other systems form the basis for predicting a small osmotic gradients in leaky epithelia. Earlier reported values of low water permeability have been criticized because of neglect of unstirred layer effects, though the validity of this criticism has also been questioned. Also, the ultrastructural techniques used to identify the putative Curran and McIntosh hypertonic middle compartment as the LIS have been criticized.
Notwithstanding these criticisms, the revised “standing gradient” model of fluid movement by local osmotic gradients still remains the most widely accepted description of water transport across intestinal epithelia.
55.2.3
Transcellular Versus Paracellular Fluid Transport
Debate on the mechanism of solute-driven water transport in near-isotonic fluid transport in leaky epithelia (small intestine and gallbladder in vertebrate GI tract) has largely focused on whether water passes through cells (transcellular) or between cells (paracellular). The revised standing-gradient model assumes that water movement is predominantly transcellular. Other models based on fluid transport secondary to solute transfer, where fluid transfer is largely paracellular, include the osmosensor feedback mechanism proposed by Hill and coworkers, and the electroosmotic model proposed by Fischbarg and coworkers (see also Ref. ). A completely different, nonosmotic mechanism, in which water is cotransported within solute transporters has been proposed by Zeuthen, Wright and coworkers. Central to the debate on the pathway of water transfer across the GI tract is the role of AQPs in fluid absorption and secretion. As discussed below, studies of AQP knockout mice have, in certain organs systems such as kidney proximal tubule, clarified the contribution of the transcellular pathway. In systems such as the small and large intestine where no role has yet been found for AQPs in physiological fluid movement, there is ongoing debate about the mechanism(s) responsible for fluid absorption and secretion ( Fig. 55.2 C).
55.2.4
Solute Recirculation
The idea of “solute recirculation” was proposed initially in the early 1990s by Ussing et al. and further explored in experiments in toad intestine. Subsequent studies confirmed and extended the initial experimental data, leading to a mathematical model of fluid absorption in which sodium recirculation accounts for absorbate isotonicity. In the proposed scheme ( Fig. 55.2 D), sodium entering into the LIS from pumping through cells or across the tight junction results in water movement. A hypertonic fluid is then extruded across the basolateral membrane of the LIS, providing the vectorial water movement component for fluid absorption. Isotonicity of the resulting absorbate is created by reuptake of sodium, putatively by the Na + /K + /2Cl − cotransporter, into cells and consequent “recirculation” back into the LIS.
The compartmental model of Larsen et al. used their data from toad intestine along with other experimental data to model transepithelial solute fluxes, initially for electroneutral absorption, and subsequently for electrogenic absorption. Their conclusions of interepithelial water flux and basolateral Na + recirculation were supported by experiments using cesium as a paracellular probe to estimate the magnitude of solvent drag in the LIS and the movement of solute from the basolateral compartment. However, the use of cesium as a paracellular tracer has been criticized, so that definitive testing of the recirculation hypothesis remains to be done. The conflicting evidence from experimental studies and modeling about whether solute movement in the LIS represents true solvent drag resulting from water flux across the tight junction, or pseudo-solvent drag resulting from solute “swept” away by water flux through the cell, emphasizes the need for further testing of solute/solvent dynamics across epithelia.
55.2.5
Water “Pumps”
An interesting hypothesis by Wright, Zeuthen, and coworkers proposes that in the small intestine (and gallbladder) water transport is mediated by “active” transport by cotransporters such as the sodium/glucose cotransporter (SGLT-1) present at the brush-border membrane of enterocytes. The theory proposes that water is cotransported stoichiometrically along with glucose and sodium as a consequence of conformational changes that occur during the normal transport cycle of SGLT-1 ( Fig. 55.2 E).
The principal evidence for active water transport comes from studies in Xenopus oocytes expressing SGLT-1, in which sodium current generated by the cotransporter is measured by microelectrodes, and cell swelling (a proxy for water transport across the membrane) is measured optically. Oocytes overexpressing SGLT-1 swell rapidly and manifest a parallel inward sodium current when exposed to an isotonic glucose-containing solution, which is inhibited by the specific blocker phlorizin. The initial fast rate of cell swelling was interpreted as the result of direct coupling of sodium and glucose transport to water transport. Additional evidence in support of this interpretation was the relatively slow rate of swelling produced by equivalent inward sodium currents generated by ion channels or ionophores. Also, it was shown that rapid changes in Na + transport driven by applied voltage changes induced concomitant changes in the rate of cell swelling. SGLT-1 has an intrinsic passive water permeability and in the steady-state sodium glucose cotransport is expected to induce osmotic water flow by changing intracellular osmolarity. It was proposed that in the steady-state that direct (nonosmotic) water cotransport contributes ~ 35% of the total water transport across the membrane. Follow-up work by Zeuthen and coworkers has implicated active water transport by a variety of cotransporters, including the Na + /glutamate cotransporter in the nervous system, H + /lactate cotransporter in retinal pigment epithelium and Na + /dicarboxylate cotransporter in kidney.
The physiological relevance of SGLT-1 water cotransport has been questioned based on studies of water transport in the kidney. Water permeability and fluid absorption in kidney proximal tubule are largely dependent on AQP1 as shown from knockout mouse studies (see below). Also, according to the proposed stochiometry of coupling of water to sodium and glucose (210:2:1, hSGLT-1), the measured quantities of sodium and glucose reabsorbed by the kidney cannot account quantitatively for the quantity of water reabsorbed. While it seems likely that cotransporter-mediated active water transport is not important in the kidney, in the small intestine it is less clear. AQP-dependent water transport in small intestine has not been demonstrated to date (see below), so in the small intestine at least, the physiological relevance of water cotransport remains an open question.
Studies by Lapointe and coworkers have called into question the interpretation and accuracy of the experimental data of Wright, Zeuthen, and coworkers in studies that aimed to replicate their findings. Lapointe and coworkers did not find good agreement between membrane voltage-induced changes in sodium current and cell swelling, concluding negligible water cotransport in the steady state. They proposed that the phenomena seen in the oocyte experiments can be accounted for quantitatively by the generation of local osmotic gradients close to the membrane (in effect, an unstirred layer), and concluded that the existing model of passive water flow across membranes produced by compartmentalized osmotic gradients can explain water transport in intestinal epithelia. Thus, there remains significant debate on details of the experimental evidence supporting the hypothesis of water cotransport, and hence water cotransport is neither sufficiently proven nor accepted to replace the established paradigm of water transport secondary to local osmotic gradients.
55.2.6
Electroosmosis
An alternative hypothesis, in which water is transported largely via a paracellular pathway, has been proposed by Fischbarg and coworkers. Although most of the experimental work that underlies the model has been carried out in the cornea, proponents of the model have suggested that it is widely applicable to fluid transporting epithelia based on previous studies showing current-induced fluid movement. The observation that underlies the model is that current across an epithelium results in fluid flows that mirror the direction and magnitude of the electrical current. In the model, active ion transport and recirculation generate a circulating current and transepithelial potential difference. The solute counterion is concurrently driven across the tight-junctions between the epithelial cells. Fluid flow across the tight junctions is consequently generated by the electroosmotic coupling of water and the solute counterion, resulting in paracellular fluid transport and isotonic fluid transport across the epithelium, although the later is dependent on the exact coupling ratio between water and counterion ( Fig. 55.2 F). The experimental basis of this theory has largely hinged on a series of studies showing current-induced fluid flow from the corneal stroma across the corneal endothelium in isolated, in vitro rabbit corneal preparations. In addition, a computational model of the corneal endothelium was developed to predict solute and fluid transport responses under experimental conditions.
Although the idea of electroosmosis has been around for many years, its application to epithelial physiology is relatively recent. In general, the theory seeks to resolve some of the apparent experimental inconsistencies in local osmosis models of fluid transport, including the quantitative difference between the reduction in osmotic water permeability (40%–50%) and the reduction in fluid transport (20%) in certain leaky epithelia in AQP knockout mouse models. Theoretical objections to electroosmosis have centered on the explanation that ion flow also produces solute concentration gradients close to the membrane, and current-induced water flow is therefore governed by local osmosis. The main counter to this argument is the fact that some experimental data show that the onset of fluid flow generated by changing transepithelial current is very fast (< 3 s) and therefore too fast to be explained by establishment of a local solute concentration gradient. Another theoretical issue is fluid flow generated by nonionic, osmotically active impermeant solutes, which cannot be explained by electroosmosis alone. Lastly, the theoretical treatment of electroosmosis is crucially dependent on number of parameters that have been only ever been tested in the cornea or are not fully described in the model. These include most crucially the coupling ratio at the tight junction, and also the composition of the tight junction charges and hydrostatic pressure effects within different epithelial compartments.
The electroosmosis model represents an interesting departure from the largely transcellular models of fluid transport and highlights the largely underappreciated importance of the tight junction in transepithelial water transport. However, the electroosmosis model has not to date been validated experimentally in different epithelia nor have its predictions been quantitatively confirmed.
55.2.7
Osmosensor Feedback Model
Hill and coworkers have proposed an interesting solution to the problem of isotonicity of transported fluid in epithelia, which relies on paracellular water flow and an osmosensor ( Fig. 55.2 G). The basis of the model is that fluid transport across an epithelium is a blend of transcellular and paracellular solute and water transport. Water is transported across the cell osmotically and is therefore largely hypertonic. Paracellular water flow is generated by forced convection of fluid through tight junctional channels that reflect solute over water, resulting in a largely hypotonic fluid. The magnitude and rate of this junctional fluid flow is proposed to be controlled by an osmosensor that samples the source solution. The fluid transported across the epithelia is osmotically clamped by the osmosensor via regulation of the rate of fluid traversing the tight junctions, resulting in a transported solution with a tonicity close to that of the source solution.
The osmosensor model as proposed relies on experimental data showing significant junctional fluid flow using dextrans as probe molecules. However, besides this indirect experimental evidence on paracellular fluid flow the model remains speculative. AQPs were proposed to be the candidate osmosensor crucial to the model, although the mechanism by which they function in this capacity has not been described nor is there any experimental evidence to support this conjecture. Further, the cellular signaling mechanisms and tight junctional apparatus involved in controlling junctional fluid transfer have not been experimentally described. Therefore, although an interesting idea to explain fluid transport in the absence of an osmotic gradient between source and sink solutions, the osmosensor model is at present a speculative, unsubstantiated theory.
55.2.8
Other Transport Theories
55.2.8.1
Countercurrent Multiplication
An intriguing model of fluid absorption, involving countercurrent exchange in the small intestinal villus, was proposed by Lundren and coworkers about 40 years ago. The idea that a countercurrent system operates in the villus was based on observations on the vascular morphology of mammalian villi. The villus contains a central arterial vessel surrounded by a dense capillary network and neighboring venous vessels. The hairpin arrangement of the arterial and venous vessels, resembling the renal microvasculature, could produce a “countercurrent” flow system in which blood flow occurs in opposite directions. The evidence that this countercurrent flow could operate as an exchange system came from measurements of relative absorption rates of the inert gases 133 Xe and 85 Kr from the intestinal lumen into the villus. These experiments, along with measurements of villus hemodynamics, suggested the presence of a villus countercurrent exchanger in several species including man.
These observations led to the proposal that the putative villus countercurrent exchanger could function as an osmotic “multiplier” as in the renal medulla. The evidence to support a multiplier function comes mainly from measurements of villus osmolality using cryoscopic techniques and sodium-sensitive microelectrodes. The villus was reported to have a large gradient of osmolality from tip to base with tip osmolality as high as 700–900 mOsm. From these measurements, it was proposed that the villus interstitium was the hypertonic compartment described in the Curran-McIntosh model. Water absorption from the intestinal lumen could be driven by the large osmotic gradient/pressure head developed in the villus tip by the countercurrent multiplier system ( Fig. 55.2 H).
The theoretical basis of countercurrent exchange and its presence in intestinal villi has been criticized (for theoretical details see Ref. ). The accuracy and validity of the techniques used to measure inert gas absorption to demonstrate countercurrent exchange have been criticized and alternative explanations for the relative gas absorption rates have been proposed. Conflicting data have also emerged on the existence of countercurrent exchange in certain mammalian species but not others, based on differences in villus anatomy. The in vitro techniques used to show high villus tip osmolality are thought to produce highly variable results, which may be an artifact of the tissue preparation. It has been pointed out that the microvascular anatomy in small intestinal villi is quite different from that of the renal medulla, and in particular there is no equivalent pathway in villi to the ascending limb of Henle in kidney. Also it has been argued that the existence of a hypertonic compartment in the villus tip does not necessarily require a countercurrent mechanism but does require a relatively water impermeable epithelium.
Thus, convincing in vivo evidence for a hypertonic villus compartment is lacking. Modern optical and fluorescent techniques may be able to elucidate the solute/solvent dynamics within the villus, although experiments on the small intestine in situ remain a significant technical challenge due to its complex geometry. Additionally, the efficiency of the putative countercurrent exchange system is critically dependent on the water permeability of the microvasculature, suggesting that endothelial AQP1 might be involved in villus fluid absorption.
55.2.8.2
Luminal Hypotonicity Driven by Acidification
An interesting model for fluid absorption in small intestine was proposed by Lucas in which the driving force for water movement across the small intestinal mucosa is located at the brush border membrane of enterocytes. This model postulates that fluid transport is driven by the neutralization of bicarbonate ions contained within an unstirred layer at the luminal membrane of enterocytes. Sodium-proton exchange at the brush border membrane produces local luminal acidification resulting from proton transport out of the cell. Bicarbonate ions diffusing from the bulk solution neutralize the protons, resulting in the production of water and carbon dioxide. The combination of two osmotically active ions to form a single osmotically active carbon dioxide molecule produces a hypotonic compartment adjacent to the luminal membrane, which, together with an increase in intracellular osmolarity from sodium entry and carbon dioxide diffusion into the cell, drives water across the membrane. Evidence for this model comes from observations of increased fluid absorption for bicarbonate-containing balanced electrolyte solutions in the upper small intestine where luminal acidification is high, along with data from bacterial diarrheas. In Salmonella typhimurium infection, it was shown that the ileum, which normally exhibits robust electrogenic Na + /glucose absorption, exhibits jejenum-like characteristics with increased luminal acidification and elevated plasma pCO 2 . This was proposed as evidence of increased Na + /H + exchange leading to fluid absorption.
Although the model suggests that the brush border membrane is the key site of solute-solvent coupling, it does not ultimately contradict the original Curran/McIntosh idea that a basolateral or abluminal hypertonic compartment drives fluid absorption. In the case of electrogenic sodium absorption by sodium channels or sodium/glucose cotransport, or absorption in the absence of luminal acidification, sodium movement across the epithelium into a putative hypertonic compartment remains the primary driving force for fluid movement. In addition, the model does not adequately explain how an osmotic gradient across the apical membrane alone results in overall fluid transport from lumen to blood, and studies of bacterially induced fluid transport argue against a significant role. Notwithstanding these caveats, the concept of a luminal hypotonic compartment potentially explains a number of unexplained observations in the upper small intestine. It remains unlikely, however, that it can explain fluid transport in other parts of the GI tract where luminal acidification does not normally occur.
55.2.9
Summary
In summary, although the general paradigm of local osmotic and hydrostatic pressure differences driving fluid transport across epithelia has been sufficient to describe measured water flows in the GI tract, a number of variations of the basic theory have been proposed as well as some completely new theories. The mechanisms involved in fluid absorption have, in general, received much more attention than the mechanisms of fluid secretion particularly in complex epithelia such as the small intestine. Secretion of saliva, bile, and pancreatic fluid can probably be explained simply and adequately by water movement into a closed/slowly moving compartment driven by osmotic gradients produced by active salt pumping. However, in small and large intestine and in gallbladder there remains significant debate on the basic water/fluid-transporting mechanisms.
55.3
Aquaporins
55.3.1
Introduction
There is an extensive body of information on the tissue localization, regulation, structure, and function of the ~ 13 mammalian AQPs identified to date. Structural studies of AQP1 indicate a homotetrameric assembly in membranes in which each monomer contains six tilted helical segments that form a barrel surrounding a water pore (reviewed in Ref. ). Functional measurements indicate that AQPs 1, 2, 4, 5, and 8 are primarily water selective, whereas AQPs 3, 7, and 9 (“aquaglyceroporins”) also transport glycerol and possibly other small solutes. Structural analysis and molecular modeling suggest that AQP water selectivity is a consequence of steric and electrostatic factors in which water moves through a narrow pore in a tortuous and single- file manner that does not permit continuous hydrogen bonding. The AQPs are expressed in many cell types involved in rapid fluid transport such as kidney tubules, exocrine gland epithelia, microvascular endothelia, choroid plexus, and ciliary epithelium. Physiological roles of AQPs have been proposed based on their tissue expression pattern and in some cases the existence of human mutations, such as nephrogenic diabetes insipidus caused by AQP2 mutation ; however, the unavailability to date of AQP inhibitors suitable for use in vivo has precluded direct investigation of their function. As described below, phenotype analysis of AQP knockout mice has elucidated specific physiological roles for many of the AQPs and has established general paradigms for their physiological roles.
55.3.2
Physiological Roles of AQPs in Nongastrointestinal Tissues
55.3.2.1
AQPs in the Kidney Tubules and the Urinary Concentrating Mechanism
The role of AQPs in kidney function has been studied extensively (reviewed in Ref. ). AQP1 is expressed in apical and basolateral plasma membranes in proximal tubule and thin descending limb of Henle and in microvascular endothelia of outer medullary descending vasa recta. AQP2 is expressed in collecting duct principal cells and undergoes antidiuretic hormone (vasopressin)-regulated trafficking between an intracellular vesicular compartment and the cell apical plasma membrane. AQP3 and AQP4 are expressed at the basolateral membrane of collecting duct epithelial cells, with relatively more AQP3 in proximal segments of collecting duct and more AQP4 in inner medullary collecting duct. Each of these AQPs plays a role in the formation of a concentrated urine. Given free access to fluid, AQP1 and AQP3 null mice were remarkably polyuric, consuming 2–3 (AQP1) to 7–10 (AQP3) times more fluid than wild-type mice, whereas the AQP4 null mice were not polyuric but manifested only a mild reduction in maximal urinary concentrating ability. After a 36-h water deprivation, urine osmolality in AQP1 null mice did not change, whereas that in AQP3 null mice increased to a submaximal level. Mutant AQP2 mice, in which a mutation causing nephrogenic diabetes insipidus in human subjects was introduced into the mouse genome, were severely polyuric and died within the first 10 days after birth. Mechanistic studies indicated that the urinary concentrating defect in AQP1 null mice results from defective proximal tubule fluid absorption and defective countercurrent multiplication producing a relatively hypoosmolar medullary interstitium. Humans with AQP1 deficiency manifest a qualitatively similar urinary concentrating defect, though maximum urine osmolality in humans (~ 1200 mOsm) is much lower than that in mice (> 3000 mOsm). Defective urinary concentrating ability in AQP2, AQP3, and AQP4 deficiency result from reduced collecting duct water permeability and consequent impaired osmotic equilibration of tubular fluid with the hypertonic medullary intestitium.
55.3.2.2
AQPs in Near-Isosmolar Fluid Absorption and Secretion
As mentioned above, impaired fluid absorption in kidney proximal tubule in AQP1 deficiency indicates the need for high transepithelial water permeability for rapid, near-isosmolar fluid transport. Indeed, micropuncture studies showed remarkable luminal hypertonicity in late proximal tubule in AQP1 null mice resulting from the retrieval of a hypertonic absorbate. An important role for AQPs was also found for near-isosmolar fluid secretion in salivary and airway submucosal gland in AQP5 deficiency, and for AQP1-dependent secretion of cerebrospinal fluid by choroid plexus and aqueous fluid by the ciliary epithelium. For example, saliva secretion was reduced by less than twofold in AQP5-deficient mice, and secreted saliva was hypertonic as a consequence of active salt secretion in the acinar lumen of salivary gland without adequate osmotic equilibration because of reduced epithelial water permeability. High transepithelial water permeability permits rapid water transport in response to active transepithelial salt transport. However, active fluid transport in many tissues does not appear to be AQP-dependent, such as alveolar fluid absorption in AQP1/AQP5 deficiency and sweat secretion in AQP5 deficiency. The rate of active fluid absorption/secretion per unit epithelial surface area in alveolus and sweat gland is much lower than that in kidney proximal tubule or salivary gland, suggesting that high AQP-dependent water permeability is not required to sustain relatively slow fluid absorption/secretion. A similar conclusion was obtained in gallbladder, where AQP1 was found to increase transepithelial osmotic water permeability by 10-fold, but not affect physiologically important gallbladder functions such as bile concentration.
55.3.2.3
AQPs in Brain Swelling and Neural Signal Transduction
AQP4 has interesting roles in the central nervous system, where it is expressed strongly throughout the brain and spinal cord, especially in astroglial cells lining ependyma and pial surfaces in contact with the cerebrospinal fluid and the blood-brain barrier. Although AQP4 null mice show no overt neurological abnormalities, they had remarkably reduced brain swelling after cytotoxic (cellular) edema produced by acute water intoxication and ischemic stroke. However, brain swelling, intracranial pressure, and clinical outcome were worse in AQP4 null mice in models of vasogenic (leaky vessel) edema, including intraparenchymal fluid infusion, cortical freeze injury, and brain tumor. Since AQP4 facilitates bidirectional water transport, its deletion in mice can thus reduce brain water accumulation in cytotoxic edema, resulting in improved clinical outcome, and reduce clearance of excess brain water in vasogenic edema, worsening clinical outcome.
Interestingly, AQP4 is also expressed in central nervous system, inner ear, and retina in supportive cells that are in close proximity to non-AQP expressing electrically excitable cells—in astroglia supporting neurons, in retinal Muller cells supporting bipolar cells, and in cochlear Clausius and Hensen’s cells supporting hair cells. Mice lacking AQP4 showed reduced seizure susceptibility and reduced evoked potential responses to light, acoustic, and olfactory stimuli. Also, mice lacking AQP4 showed remarkable retinal neuroprotection after ischemic-reperfusion challenge. Based on AQP4 and K + channel Kir4.1 colocalization, it has been proposed that AQP4 in supportive cells may facilitate K + recycling during electrical signal transduction in brain, retina, and inner ear. While AQP4 does not directly modulate the activity of Kir4.1, there may be alterations in extracellular space dynamics in AQP4 deficiency as a consequence of saltwater coupling. In support of this possibility, we found using microfiberoptic fluorescence photobleaching and ratio imaging methods that the extracellular space was expanded in cerebral cortex in AQP4-deficient mice. Quantitative studies relating extracellular space volume and ionic concentration to neural stimuli are needed to establish the mechanism of altered neural signal transduction in AQP4 deficiency. In the GI tract, AQP1 has been localized to glial cells in mesenteric and pancreatic nerve ganglia, though its function in the peripheral nervous system remains unknown.
55.3.2.4
AQPs in Corneal Edema and Transparency
Studies of corneal swelling suggest yet another interesting role for AQPs. Maintenance of corneal transparency requires precise regulation of stromal water content. AQP1 is expressed in corneal endothelial cells and AQP5 in epithelial cells. Corneal thickness, water permeability, and response to experimental swelling in wild-type mice and mice lacking AQP1 or AQP5. Compared to wild-type mice with corneal thickness of 123 μm, corneal thickness was reduced in AQP1 null mice (101 μm) and increased in AQP5 null mice (144 μm). After exposure of the external corneal surface to hypotonic saline (100 mOsm), the rate of corneal swelling (5 μm/min) was reduced approximately twofold by AQP5 deletion. After exposure of the corneal endothelial surface to hypotonic saline by anterior chamber perfusion, the rate of corneal swelling (7 μm/min) was reduced approximately fourfold by AQP1 deletion. Although baseline corneal transparency was not impaired by AQP1 deletion, the recovery of corneal transparency and thickness after hypotonic swelling (10 min exposure of corneal surface to distilled water) was remarkably delayed in AQP1 null mice, with ~ 75% recovery at 7 min in wild-type mice compared to 5% recovery in AQP1 null mice. The impaired recovery of corneal transparency in AQP1 null mice provides evidence for the involvement of AQP1 in active extrusion of fluid from the corneal stroma across the corneal endothelium.
55.3.2.5
Roles of Aquaglyceroporins—AQP3 in Skin Hydration and Cell Proliferation, and AQP7 in Fat Cells
The water/glycerol transporting protein AQP3 is expressed strongly in epidermal keratinocytes, which form the interface between the dermis/vasculature and the stratum corneum. The hypothesis that AQP3 is an important determinant of skin hydration and barrier/mechanical properties was tested by comparative measurements in wild-type and AQP3 null mice generated in a hairless genetic background. High-frequency skin conductance, a direct measure of stratum corneum hydration, was remarkably (approximately twofold) reduced in the AQP3 null mice, and this differences persisted even when mice were placed in a humidified atmosphere or when the skin was covered by an occlusive dressing. Transepidermal water loss measurements after tape-stripping revealed significantly slowed recovery of barrier function in the AQP3 null mice. Skin elasticity, measured from the kinetics of skin displacement in response to rapidly applied suction, was remarkably reduced in the AQP3 null mice due to abnormal stratum corneum mechanical properties; skin elasticity of wild type and AQP3 null mice became similar after removal of the stratum corneum by tape-stripping. AQP3 thus plays an important role in skin hydration and barrier/mechanical properties. A detailed comparison of skin morphology, and the composition of the stratum corneum and epidermis revealed reduced glycerol content as the main abnormality in AQP3 deficiency. The various abnormalities in stratum corneum hydration, barrier recovery, and skin elasticity could be related to reduced glycerol content based on the humectant (water-retaining) and biosynthetic functions of AQP3. In addition, topical or systemic glycerol replacement corrected the skin abnormalities in the AQP3 null mice, supporting the conclusion that the glycerol-transporting function of AQP3 is important for skin hydration and biosynthetic functions.
In addition to epidermal keratinocytes, AQP3 is also expressed in corneal and colonic epithelium. We discovered a novel role for AQP3 in cell proliferation in studies of wound healing and tumorigenesis in skin, corneal surface repair, and colonic regeneration. AQP deficiency was associated with slowed healing of cutaneous and corneal wounds, with reduced cell proliferation as measured by BrdU incorporation. Motivated by these results supporting the involvement of AQP3 in cell proliferation, we discovered a remarkable phenotype using a skin tumor model of epidermal cell proliferation in which cutaneous papillomas were produced by repeated inducer-promoter exposure. Mice lacking AQP3 failed to produce papillomas following the same treatment in which all wild-type mice produced multiple papillomas. Studies in various intact skin and keratinocyte culture models suggested that AQP3-facilitated glycerol transport is a key determinant of cell proliferation by a mechanism involving reduced epidermal glycerol concentration in AQP3 deficiency, resulting in impaired lipid biosynthesis and reduced glycerol metabolism and ATP, with consequent impairment in MAP kinase signaling and reduced cell proliferation. AQP3-dependent cell proliferation probably has relevance to epithelial regeneration in colitis, as discussed further below, and for the growth and proliferation of some GI tumors.
AQP7, which is expressed strongly in adipocyte plasma membranes, also functions as water/glycerol transporting protein. We found remarkable age-dependent adipocyte hypertrophy in AQP7-deficient mice and attributed the phenotype to defective AQP7-dependent glycerol transport. Wild type and AQP7 null mice had similar growth at 0–16 weeks as assessed by body weight, though by 16 weeks AQP7 null mice had 3.7-fold increased body fat mass. Adipocytes from AQP7 null mice of age 16 weeks were greatly enlarged (diameter 39 μm) compared to wild-type mice (118 μm). Adipocytes from AQP7 null mice also accumulated excess glycerol (251 vs. 86 nmol/mg protein) and triglycerides (3.4 vs. 1.7 μmol/mg protein). In contrast, at the age of 4 weeks, adipocyte volume and body fat mass were comparable in wild type and AQP7 null mice. To investigate the mechanism(s) responsible for the progressive adipocyte hypertrophy, glycerol permeability and fat metabolism were studied in adipocytes isolated from the younger mice. Plasma membrane glycerol permeability measured by 14 C-glycerol uptake was threefold reduced in AQP7-deficient adipocytes. However, adipocyte lipolysis, measured by free fatty acid release and hormone-sensitive lipase activity, and lipogenesis, measured by 14 C-glucose incorporation into triglycerides, were not affected by AQP7 deletion. The results supported the conclusion that adipocyte hypertrophy in AQP7 deficiency results from defective glycerol exit and consequent accumulation of glycerol and triglycerides, suggesting the possibility of pharmacological upregulation of adipocyte AQP7 expression/function to reduce adipocyte size and fat mass in obesity (reviewed in Ref. ).
55.3.2.6
AQPs in Angiogenesis and Cell Migration
We discovered a new function of AQPs in facilitating cell migration. The original motivation for studying the role of AQPs in angiogenesis was the observation of strong AQP1 expression in tumor microvessels. Remarkably, impaired tumor growth was found in AQP1 null mice after subcutaneous or intracranial tumor cell implantation, with reduced tumor vascularity and extensive necrosis. Reduced microvessel growth was also found in implanted pellets of Matrigel-containing vascular endothelial growth factor. A novel mechanism for the impaired angiogenesis was established from cell culture studies. Although adhesion and proliferation were similar in primary cultures of aortic endothelia from wild type versus AQP1 null mice, cell migration was greatly impaired in AQP1-deficient cells, with abnormal vessel formation in vitro. Stable transfection of nonendothelial cells with AQP1, or a structurally different water-selective transporter (AQP4), accelerated cell migration and in vitro wound healing. Interestingly, the migrating AQP1-expressing cells had prominent membrane ruffles at the leading edge with polarization of AQP1 protein to lamellipodia, where rapid water fluxes occur. As a possible mechanism for the dependence of cell migration on AQPs, we proposed that cell membrane protrusions are formed as a consequence of actin cleavage and ion uptake at the tip of a lamellipodium, creating local osmotic gradients that drive the influx of water through the cell membrane. Water entry would then increase local hydrostatic pressure to cause cell membrane protrusion, resulting in forward cell movement. The presence of an AQP in lamellipodia would augment this process.
AQP-facilitated cell migration appears to be a general phenomenon that is not cell type or AQP specific (reviewed in Ref. ). For example, we have characterized AQP4-dependent cell migration in kidney proximal tubule cells, AQP4-facilitated migration of astroglia cells in brain and AQP3-facilitated migration of epidermal keratinocytes and corneal epithelial cells. A recent study reported circumstantial evidence that AQP1 facilitates pathological angiogenesis in cirrhosis. Of particular interest was the observation that AQP expression in tumor cells enhanced their migration, metastatic potential, and local invasiveness. Many tumors express AQPs and in some cases AQP expression correlates well with tumor grade (reviewed in Ref. ). The enhancement of tumor invasion by AQPs suggests AQP inhibition as a new approach for tumor therapy.
55.3.2.7
Aquaporins and Hydrogen Peroxide Transport
Recent evidence indicates that some AQPs are able to transport hydrogen peroxide (H 2 O 2 ) in addition to water. Early studies investigating the functional significance of AQP-facilitated H 2 O 2 transport showed that loss of AQP3-mediated H 2 O 2 transport impaired chemokine-stimulated T-cell migration. The development of psoriasis and TNFα/NFκB signaling in skin keratinocytes was shown to be dependent on AQP3-facilitated H 2 O 2 transport. Other studies showed the involvement of AQP3-dependent H 2 O 2 transport in breast cancer cell migration and epidermal growth factor signaling. In the colon (see below), AQP3-mediated H 2 O 2 transport was recently shown to modulate key epithelial innate immune responses. Because H 2 O 2 is an important and emerging intra- and extracellular signaling molecule, AQP-dependent H 2 O 2 signaling responses may be important in many cells and tissues.
55.3.3
General Paradigms About Physiological Functions of AQPs
In summary, studies of the extra-GI phenotype of AQP-deficient mice suggest that AQP-facilitated water permeability is important: (a) when water movement is driven across a barrier by a continuous osmotic gradient (as in kidney collecting duct); (b) for active, near-isosmolar fluid absorption/secretion (as in kidney proximal tubule and salivary gland); (c) for neural signal transduction (as in brain and inner ear); and (d) for cell migration (as in tumor vessel angiogenesis). Glyercol transport by the aquaglyceroporins is involved in skin hydration and cell proliferation (AQP3) and adipocyte metabolism (AQP7). AQP-dependent H 2 O 2 transport is involved in signaling response in skin, immune cells, colonic epithelium, and potentially other tissues. Another conclusion from the phenotype studies is that the tissue expression of an AQP does not ensure its physiological significance, so that evaluation of tissue AQP function must be done on a case-by-case basis. For example, despite the expression of AQP4 in skeletal muscle, no phenotype abnormalities in AQP4 null mice were found, as was the case for several AQPs in airways and lung. From these general paradigms, a variety of diverse roles of AQPs in the GI tract are possible, as discussed below.
55.3.4
Aquaporin Expression in the GI Tract
The expression of specific AQPs in the GI tract provides clues to possible functional roles. Several well-documented expression patterns provide indirect evidence for a possible role of AQPs in these tissues, including: AQP1 in intrahepatic bile duct epithelium (bile formation), intestinal lacteals (fat absorption), and microvascular endothelia throughout the GI tract (fluid absorption/secretion); AQP4 in basolateral membrane of parietal cells in stomach (acid/fluid secretion) and colon surface epithelium (fluid absorption); AQP5 in apical membrane of acinar cells in salivary glands (saliva secretion); AQP8 in salivary gland, liver, pancreas, and intestine; and AQP9 in hepatocytes. Fig. 55.3 shows examples of AQP protein localization in the GI tract, with AQP1 in central lacteals of small intestine (A), AQP4 at the basolateral membrane of surface epithelial cells in colon (B), AQP8 in crypt epithelium in ascending colon (C), and AQP4 at the basolateral membrane of gastric parietal cells (D). Additional information about the localization of these and other AQPs is discussed below, together with information about organ-specific fluid transporting mechanisms and available data from AQP knockout mice.

55.4
Fluid Transport Mechanisms and Aquaporins in GI Organs
55.4.1
Salivary Gland
Saliva secreted by the salivary gland is the first fluid with which ingested food comes into contact. The interstitial-to-luminal transport of sodium and chloride across the acinar epithelium is the driving force for osmotic water movement. The salivary duct epithelium is believed to be relatively water impermeable, in which the sodium and chloride are absorbed and potassium and bicarbonate secreted to produce a hypotonic saliva. Upon stimulation, the salivary gland can secrete saliva at high rates (up to 50 mL/min/100 g tissue in humans), which relies on rapid water movement from serosa-to-mucosa across the capillary endothelium and acinar cells. The possible involvement of AQPs in this process has been proposed based on the expression of AQP1 in microvascular endothelial cells of salivary gland, AQP5 in apical membrane of acinar cells, and AQP8 in acinar cells. A more recent study of expression of AQPs 1, 3, 4, and 5 in human salivary gland reported AQP1 in microvessels and AQP5 at the apical membrane of acinar cells as found in rodent salivary gland, as well as AQP3 at the basolateral membrane of both serous and mucous acinar cells.
Our laboratory generated AQP5 null mice to investigate the role of AQP5 in saliva secretion. Pilocarpine-stimulated saliva production was reduced by > 60% in AQP5 null mice. Compared with the saliva from wild-type mice, the saliva from AQP5 null mice was hypertonic (420 mosM) and remarkably more viscous ( Fig. 55.4 ). Amylase and protein secretion and functions of salivary mucous cells were not affected by AQP5 deletion. Saliva secretion was not impaired in AQP1 or AQP4 knockout mice. A subsequent report confirmed the defect in saliva secretion in AQP5 null mice and reported reduced water permeability, as expected, in acinar cells isolated from the null mice. In a follow-on study, no effect was found of AQP8 deletion on saliva secretion in mice, nor was there a difference in saliva secretion in AQP8/AQP5 double knockout mice compared to AQP5 knockout mice.

One study has suggested that AQP5 has an abnormal intracellular distribution in Sjogren’s syndrome subjects, though another study reported the opposite finding. Another immunolocalization study in rat showed that AQP5 in salivary gland acinar cells is primarily at the apical plasma membrane under both basal and stimulated conditions. Although there may be a subset of subjects with Sjogren’s syndrome with cellular mislocalization of AQP5, it is more likely that AQP5 mislocalization, if it occurs, is a consequence of the chronic immune damage to the salivary gland.
There has been considerable interest in the possibility of AQP gene therapy to treat salivary gland dysfunction following radiation therapy-induced injury. Adenoviral transfer of human AQP1 to mice following head and neck irradiation showed increased saliva secretion and water permeability in isolated acinar cells. Introduction of AQP1 by adeno-associated virus in irradiated mini-pigs also showed increased saliva secretion, and early-phase human clinical trials involving adenoviral transfer of AQP1 by retrograde infusion into parotid glands suggested potential benefit. However, it remains to be determined whether there will be long term, clinically significant benefit of AQP gene transfer to hypofunctioning salivary glands, particularly with repeated AQP gene administrations and when gland tissue is largely destroyed.
55.4.2
Stomach
The large quantity of gastric fluid produced by the mammalian stomach is thought to be secreted mainly by fundic glands in the mucosa of the stomach body. These glands contain mucus cells, chief cells, and parietal cells that secrete mucus, pepsinogen, and hydrochloric acid, respectively. During agonist-stimulated acid secretion in humans, gastric juice is transported from the mucosal interstitium into the gastric lumen at a rate of ~ 0.7 mL/min/100 g tissue. There is little information about the relative contributions of different cell types involved in gastric fluid secretion. So far, AQP4 is the only AQP identified in the stomach. Rat AQP4 was immunolocalized to the basolateral membrane of parietal cells. A human AQP4 homolog was subsequently cloned from stomach and immunolocalized to both parietal cells and chief cells. A more recent evaluation of AQP4 expression in gastric glands showed AQP4 protein localization only in parietal cells in mid and deep regions of gastric glands. One interesting study addressed the possible importance of AQP4 assembly in orthogonal arrays of proteins (OAPs), which are lattice-like particle arrangements seen by freeze-fracture electron microscopy. AQP4 was shown to be the main component of OAPs from studies in AQP4-expressing CHO cells and from the absence of OAPs in multiple tissues in AQP4-deficient mice. Carmosino et al. reported that OAPs in an AQP4 transfected human gastric cell line was reduced by ~ 50% after histamine stimulation suggesting that short-term regulation of AQP4 assembly and water permeability might be possible. However, the physiological significance of this observation is unclear.
One study investigated the role of AQP4 in gastric acid and fluid secretion utilizing AQP4 null mice. Gastric acid secretion was measured in anesthetized mice in which the stomach was lumenally perfused with normal saline-containing 14 C-PEG as a volume marker. Collected effluent was assayed for titrable acid content and 14 C-PEG radioactivity. No significant differences were found between wild type and AQP4 null mice in baseline gastric acid secretion, in pentagastrin stimulated acid secretion, or in histamine-/carbachol-stimulated acid secretion, nor did AQP4 deletion affect gastric fluid secretion, gastric pH, or fasting serum gastrin concentration. Thus, the knockout mouse data did not show a physiological role of AQP4 in the stomach.
55.4.3
Liver
The expression of several AQPs (1, 3, 8, and 9) has been reported in liver and proposed to play a role in bile secretion. Early immunocytochemistry showed AQP1 expression on the apical and basolateral membranes of cholangiocytes. Work by the LaRusso group suggested a potential role of several AQPs in bile secretion. Semiquantitative water permeability measurements in isolated cholangiocytes and intrahepatic bile ducts suggested AQP-mediated water transport based on weakly temperature-dependent water transport and inhibition by HgCl 2. Membrane fractionation studies suggested that secretin caused a redistribution of AQP1 from intracellular vesicles to the cell plasma membrane, and that the redistribution was blocked by colchicine and low temperature. If correct, these observations would indicate that unlike other cell types where AQP1 is expressed constitutively at the plasma membrane, the cholangiocyte possesses a unique regulatory mechanism for AQP1. However, the cell biology of regulated AQP1 trafficking will require elucidation, since AQP1 (unlike AQP2 ) does not contain a consensus phosphorylation site at its C-terminus. Immunocytochemistry in rat and mouse showed AQP8 protein expression in intracellular vesicles in hepatocytes. Further measurements of water permeability in isolated hepatocytes from rat showed moderate water permeability ( P f ) of 66 × 10 − 4 cm/s at 37°C ; however, follow-up studies from the same group reported a much lower P f of < 10 − 4 cm/s. They also reported a cAMP agonist-induced ~ 2-fold increase in rat hepatocyte water permeability, relocalization of intracellular AQP8 to the plasma membrane, and a sixfold increase in water permeability of canalicular plasma membrane domains in cAMP-treated rat hepatocytes. Studies using AQP8 knockout mice as control showed AQP8 immunolocalization in the plasma membranes of hepatocytes in mice with weak intracellular labeling. Osmotic water permeability measured in freshly isolated hepatocytes from wild-type mice was low ( P f 6 × 10 − 4 cm/s) and not increased after cAMP agonists or reduced in AQP8 deficiency, providing evidence against constitutive or cAMP-regulated AQP8 water permeability in hepatocytes in mice. As is the case for AQP1, rat and mouse AQP8 do not contain consensus sequences for phosphorylation by protein kinases A or C. An interesting role of AQP1 in hepatic angiogenesis in cirrhosis was reported in which AQP1 knockdown impaired migrating endothelial cells during angiogenesis.
Whether AQPs facilitate bile secretion remains unclear. Measurements in AQP1 and AQP8 knockout mice do not support a significant role of AQPs in bile secretion in mice. Fluid collections by ductal cannulations in adult mice showed no effect of AQP1 deletion on bile flow rate and bile salt concentration. Secretin/CCK produced a threefold increase in pancreatic fluid flow, though fluid volumes, pH and amylase activity were not affected by AQP1 deletion. Also, direct measurements of water permeability and fluid secretion in cultures of mouse cholangiocytes do not support a significant role of AQP1 in bile secretion. Limited studies done on AQP8 null mice stressed by a high fat diet to expose potentially subtle defects in hepatobiliary function. Weight gain in wild type and AQP8 null mice on a high fat diet was similar, and the AQP8 null mice did not develop steatorrhea or abnormalities in serum lipid profile, liver function tests, or pancreatic enzymes. It was concluded that AQP8 does not have an essential role in hepato/biliary/pancreatic function, though a more definitive conclusion will require direct measurements on bile and pancreatic fluid. In a cell culture study, AQP8 knockdown in HepG2 cell cultures was associated with impaired canalicular water handling, though it is difficult to extrapolate results from cell culture models to bile secretion in vivo. Another cell culture study involving AQP8 knockdown in rat hepatocytes suggested the involvement of AQP8 in mitochondria in ammonia detoxification by facilitating ammonia entry into mitochondria.
Despite evidence largely against involvement of AQPs in bile secretion, there has been interest in AQP gene delivery for cholestasis. Marrone et al. reported that delivery of an adenovirus encoding AQP1 by retrograde infusion into the bile duct increased bile flow in an estrogen-induced rat model of cholestasis. The logic was that increasing water permeability of the hepatocyte canalicular membrane would increase osmotically driven water secretion from hepatocytes into bile canaliculi. However, these studies have been criticized based on arguments related to the small size and high surface-to-volume ratio of bile canaliculi, the patchy nature of AQP1 expression following gene transfer, and difficult-to-explain effects on bile salt secretion. If gene therapy of cholestasis is to be pursued, it would seem more logical to deliver genes encoding bile salt transporters/pumps to increase bile flow.
Several studies have reported AQP1 expression in pancreatic ducts and/or pancreatic microvasculature ; however, the unimpaired pancreatic fluid secretion in AQP1 null mice raises doubt about the physiological significance of pancreatic AQP1 expression, at least in mice. AQP5 and AQP8 expression in pancreas were also found in several expression studies, though direct functional studies in knockout mice have not been done.
There is evidence for possible involvement of AQP9 in metabolism-related hepatocyte functions. AQP9 appears to be a relatively nonselective AQP that transports water, glycerol and a variety of other small polar solutes. Knockout mice lacking AQP9 manifest a defect in hepatic glycerol update, with increased plasma glycerol and triglycerides in AQP9 null mice, and abnormal blood glucose regulation in a strain of obese mice into which was introduced the AQP9 gene deletion. Studies in hepatocyte cultures and perfused livers showed that AQP9 is the primary route for glycerol entry into hepatocytes in glycerol gluconeogenesis ; the same group reported that AQP9 together with a type-A urea transporter facilitated urea entry into hepatocytes. An interesting alternative role for AQP9 in hepatocytes has been suggested based on the observation of reduced arsenic clearance and increased arsensic toxicity in AQP9 knockout mice. Mice administered sodium arsenite showed arsenic accumulation in liver with reduced fecal and urinary excretion, suggesting that hepatocyte AQP9 is involved in arsenic exit from liver. However, it remains unclear whether the observed phenotype is a direct consequence of AQP9 gene deletion in hepatocytes or a secondary phenomenon related to alterations in other hepatocyte genes involved in arsenic detoxification in AQP9 deficiency.
55.4.4
Gallbladder
The gallbladder epithelium is perhaps the most intensely investigated area of the GI tract in relation to water transport, in part because it is relatively easy to study, consisting of a simple, relatively flat epithelium with large cuboidal cells that are homogenous in size and functional properties. Also, the gallbladder is a blind sac allowing assessment of water transport by relatively simple gravimetric techniques. Studies on rabbit gallbladder formed the original experimental basis for the “standing gradient” model of fluid absorption in leaky epithelia. Follow-up studies in mammalian and Necturus gallbladder investigated the osmotic water permeability and morphology of the apical (lumen-facing) membrane and the epithelium as a whole in an effort to measure the primary parameters to differentiate among the various fluid transport models for transepithelial fluid transport.
The gallbladder is primarily an absorptive epithelium that functions to concentrate sodium salts of bile acids by near-isotonic fluid absorption from the gallbladder lumen. Transcellular water flux across highly water permeable apical and basolateral cell membranes is the prevailing view on the mechanism of fluid absorption across the gallbladder epithelium. However, this view has been questioned based on both methodological and theoretical considerations (see Epithelial Transport Mechanisms section). AQP1 is prominently expressed on the apical plasma membrane of gallbladder epithelial cells. A possible role for AQP1 in mouse gallbladder was investigated using knockout mice. Transepithelial osmotic water permeability ( P f ) was measured in freshly isolated gallbladder sacs from the kinetics of luminal calcein self-quenching in response to an osmotic gradient. P f was very high (0.12 cm/s) in gallbladders from wild-type mice and reduced by ~ 10-fold in AQP1 null mice, despite similar gallbladder morphology. However, neither bile osmolality nor bile salt concentration differed in gallbladders from wild type versus AQP1 knockout mice. The data indicated constitutively high water permeability in mouse gallbladder epithelium involving transcellular water transport through AQP1. However, the similar bile salt concentration in gallbladders from AQP1 knockout mice provides evidence against a physiologically important role for AQP1 in mouse gallbladder.
55.4.5
Small Intestine
The normally functioning small intestine carries out substantial net absorption of fluid (~ 6–7 L per day in humans), which represents a combination of absorptive and secretory fluid fluxes. In response to various stimuli and in disease states such as bacterial/viral infection, net fluid absorption switches to fluid secretion that can result in diarrhea. The small intestine is a geometrically complex epithelia with many villi protruding into the intestinal lumen, thereby increasing the effective surface area of the epithelium for solute and water absorption. Interspersed between the villi are the “gland like” crypts of Lieberkuhn that form the progenitor cells for the villus tips. It has been postulated that fluid secretion occurs predominantly within the crypt cells and fluid absorption at the villus tip. This view has begun to be revised in recent years with both absorptive and secretory functions being ascribed to cells in both locations.
The magnitude of fluid movement varies along the length of the small intestine with the bulk of fluid absorption occurring in the jejunum and ileum. As discussed in detail in the “Fluid Transporting Mechanisms” section, the mechanism and pathway of water transport across the small intestinal epithelium remain unresolved.
Expression of at least eight AQPs in small intestine has been reported : AQP1 in endothelia and lacteals of small intestine and microvascular endothelia throughout the intestine; AQP3 at the basolateral membrane of the epithelial cells lining the villus tip of the small intestine in rat; AQP4 at the basolateral membrane of crypt epithelium; AQP5 in the apical membrane of secretory cells in duodenal glands; AQP6 in surface epithelial cells, AQP8 at apical membrane of epithelia in rat small intestine; and AQP9 in goblet cells in duodenum, jejunum, ileum. Another water channel, AQP10, has been identified with transcript expression in human duodenum and jejunum, and protein expression in the apical membrane of villus epithelium in ileum.
55.4.5.1
Duodenum
A primary role of the duodenum is the protection of epithelium and underlying tissue from damage by gastric acid and proteases produced in the stomach. The duodenum achieves this primarily by the secretion of mucus and bicarbonate into the lumen from the epithelium and submucosal glands (Brunner’s glands) forming a protective mucus-bicarbonate barrier. The mucus barrier is formed when mucins and water combine to form a bicarbonate-rich viscoelastic gel that creates a physiochemical barrier to acid and enzymes.
Secretion of mucus fluid from Brunner’s glands and the epithelium clearly has an important role in normal duodenal function. However, the pathway for the water component of this secretion is so far undetermined. AQP5 has been found at the apical membrane of acinar cells in Brunner’s glands, suggesting a possible role in fluid secretion from glands. There is no functional data at presence for facilitated water transport in duodenum.
55.4.5.2
Jejenum and Ileum
Water movement in the jejenum and ileum in the healthy intestine is primarily absorptive. Bacterial toxins such as cholera toxin and Escherichia coli STa toxin act on enterocytes resulting in massive secretion of NaCl and a consequent reversal of net water flux from absorptive to secretory, producing diarrhea. The role of AQPs in fluid absorption in the jejenum and ileum is at present unclear. Osmotic water permeability of the apical membrane of small intestinal enterocytes, when measured minimizing confounding effects of unstrirred layers, is generally found to be fairly high. Early perfusion studies suggested that proximal segments of small intestine have higher osmotic permeability than distal segments. The proximal jejunum has been proposed to be highly water permeable to permit rapid osmotic equilibration of intestinal contents. It has been therefore proposed that (see Standing-gradient model) the transcellular water permeability is sufficiently high to account for the majority of water transport across the epithelium. Whether AQPs are involved in this process is unclear. One possibility is that the specialized lipid composition of enterocyte plasma membranes and their convoluted microvillar ultrastructure confer high transcellular water permeability in the absence of AQPs.
Another widely accepted model states that water moves across the epithelium primarily by a paracellular route across the tight junctions between neighboring cells. Since the small intestinal epithelium has low electrical resistance, low reflection coefficients for small solutes, and high paracellular solute flux, it has proposed that the tight junctions are also permeable to water. Small intestinal tight junctions have thought to be cation selective and permeable to large polar nonelectrolytes. This led to the suggestion that osmotic gradients created by active transport of solutes along with the passive ion permeability through the tight junction results in “solvent drag” of water through the paracellular pathway (see also Sodium Recirculation). Several experimental and theoretical studies based on the dynamics of various paracellular probes have supported the paracellular model for water movement, the interpretation of the data is still subject to considerable debate. An alternative pathway that has been proposed is that transport occurs via the SGLT cotransporter (see Water Pumps).
Experiments on AQP knockout mice have found little difference in fluid absorption or secretion in the small intestine compared to wild-type mice. At least four AQPs have been reported in the epithelial cells; AQP4 in the basolateral membrane of enterocytes at the base of crypts in the ileum, AQP3 in the basolateral membrane of villus enterocytes, AQP8 in the apical membrane of both crypt and enterocytes, and AQP10 at the apical membrane of enterocytes in ileum. AQP4 is only expressed at the very base of crypts in undifferentiated enterocytes and so thought not to be responsible for fluid transport in the intestine. Also, it is unclear whether AQP3 is actually expressed in small intestinal enterocytes with equivocal immunostaining reported in tissues. AQP8 may be the main AQP expressed in small intestinal crypt cells. However, analysis of AQP8 null mice showed little phenotypic differences compared to wild-type mice. Osmotically driven water secretion, isosmolar fluid absorption, and cholera toxin-induced active fluid secretion did not differ significantly in wild type versus AQP8 null mice.
55.4.6
Colon
Water transport in the colon is primarily absorptive and occurs against a significant osmotic and hydraulic resistance imposed by the luminal contents. The primary functions of the colon are the salvage of the remaining fluid and electrolytes entering from the small intestine and the dehydration and storage of feces. The colon is a tight epithelium with substantially higher electrical resistance than the small intestine and a much lower paracellular permeability. The colonic epithelium consists of a flat surface epithelium and numerous deep crypts containing absorptive and secretory columnar epithelial cells. Additionally, colonic structure and function vary along its length with fecal dehydration becoming more important distally. Early studies showed that in contrast to the small intestine the colon is able to transport fluid hypertonically, with absorbate osmolalities of 500–1000 mOsm. As in the small intestine the route of water movement in the colon has been the subject of ongoing debate. At least three AQPs are expressed in the colon—AQP3 and AQP4 in surface epithelial cells and AQP8 in crypt epithelial cells. There are reports of AQP8 upregulation in human colon in ulcerative colitis and downregulation in a mouse model allergic diarrhea, though there is no evidence for involvement of AQP8 in the pathophysiology of these processes.
Studies in AQP knockout mice have not demonstrated a significant role for AQPs in colonic fluid absorption. AQP4 is localized at the basolateral membrane of surface colonic epithelial cells with strongest expression in proximal colon. Though a small increases in stool water content were found in AQP4 knockout mice and a small reduction in osmotic water permeability in proximal (but not distal) colon, there was no impairment of the marked colonic fluid secretion induced by theophylline. In AQP8 knockout mice, no differences were seen comparing to wild type mice in isosmolar fluid absorption in closed loops of descending colon or in stool water content. We note, however, several recent studies showing drug effects on intestinal fluid transport and AQP expression, though it was not determined when changes seen in AQP expression were a primary drug action or secondary to altered intestinal physiology. Recent studies of AQP3 inhibition by rectal administration of mercury or copper ions reported development of diarrhea, though the diarrhea was more likely the consequence of metal ion toxicity than of AQP3 inhibition.
The apparently minimal role of AQPs in water absorption in the colon suggests that other mechanisms are involved. A significant proportion of water absorption occurs at the surface epithelium of both proximal and distal colon although the exact route of water flux is unclear. The process of fecal dehydration occurs against substantial osmotic and hydraulic resistance imposed by feces as their water content is reduced from 95% to 70%. The mechanism by which the colon carries out fecal dehydration is not yet resolved. Several studies have suggested that colonic crypts are the major site of both fluid absorption and agonist-stimulated fluid secretion. Studies by Naftalin et al. using agarose gel cylinders to mimic the hydraulic resistances imposed by feces, as well as optical studies using fluorescent dextrans, indicated that colonic crypts play an important role in colonic fluid absorption. These studies led to a model of fecal dehydration, based on the original Curran and McIntosh model, in which solute absorption by colonic crypts produces a hypertonic pericryptal compartment outside of the crypt lumen ( Fig. 55.5 ). Distal colonic crypts are surrounded by a fenestrated membrane or “sheath” consisting of myofibroblast cells and extracellular matrix components, creating a diffusion barrier to sodium and providing mechanical rigidity to resist collapse of the crypt under negative pressure. Experiments using a fluorescent sodium indicator showed high sodium concentrations in the pericryptal space. The resultant osmotic gradient is proposed to drive water transport from the crypt lumen across a relatively water impermeable barrier, allowing the mouth of the crypt to act as a suction device to absorb water out of fecal material within the colon lumen. Initial evidence for this mechanism was the demonstration by photobleaching experiments of convective fluid flow within crypts. Additional evidence for a role for the “pericryptal sheath” in modulating fluid absorption comes from studies showing that proabsorptive stimuli, such as high plasma rennin-angiotensin II-aldosterone, correlate with a trophic effect on the pericryptal sheath and increased pericryptal sodium concentrations. However, though the suction hypothesis is currently the only mechanism proposed to account for fecal dehydration, direct measurements of crypt suction and water permeability are needed to investigate this interesting mechanism further.
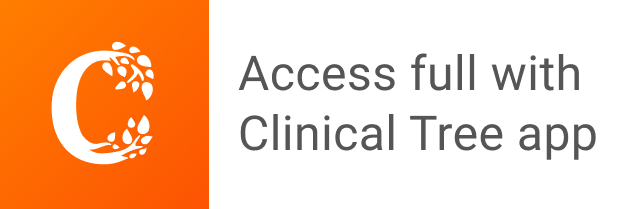