Study
Model
Diet
Renal function
Osteochondrocytic marker(s)
Arterial mineralization
Location
Mechanism
Luo et al. [82]
Mgp−/− mice
Standard
Normal
↑Col II
Medial
Chondrocytic
Davis et al. [218]
Ldlr−/− mice
High fat
STX (uremic)
↑OCN
Intimal
Osteoblastic
Bobryshev [219]
ApoE−/− mice
Standard
Normal
↑Sox9, Col II
Intimal
Chondrocytic
Rattazi et al. [220]
ApoE−/− mice
Standard
Normal
↑Col II, OPN, ALP
Intimal
Chondrocytic
Al-Aly et al. [78]
Ldlr−/− mice
High fat
Normal
↑BMP2, Runx2, Msx2, Wnt3a/7d
Intimal/Medial
Osteoblastic
Neven et al. [79]
Wistar rats
High P/low protein
Adenine-induced CRF
↑Sox9, Runx2, Col II
Medial
Chondrocytic
Kawata et al. [221]
Sprague–Dawley rats
High P
STX (uremic)
↑Runx2, OCN, OPN, MGP
Medial
Osteoblastic
El-Abbadi et al. [80]
DBA/2 mice
High P
STX (uremic)
↑Runx2, OPN; ↓SM22α (no Col II staining)
Medial
Osteoblastic
Koleganova et al. [222]
Sprague–Dawley rats
Standard
STX (uremic)
↑Runx2, OSN, BMP2 (MGP, OPN, Pit1: ns vs. sham)
Intimal/Medial
Osteoblastic
Speer et al. [83]
Mgp−/− mice
Standard
Normal
↑Runx2, OPN, Col II; ↓Msx2, myocardin; Osx, Wnt3a/7d
Medial
Osteochondrocytic
Undetected; (Sox9, BMP2: ns vs. Mgp+/+)
Neven et al. [79]
Wistar rats
High P/low protein
Adenine-induced CRF
↑Runx2, Sox9, Col II, aggrecan; ↓Osx, LRP6, β-catenin
Medial
Chondrocytic
Doehring et al. [223]
Ldlr−/− mice
High fat
Normal
↑Sox9, Col II
Intimal
Chondrocytic
3.1.2 Technical Challenges in Identifying Phenotypically Modulated VSMC Present in Atherosclerotic Lesions In Vivo
In the context of atherosclerotic lesions the extent of the contribution of VSMC to mineralization is not clear due to difficulties in definitively identifying phenotypically modulated cells. Mature VSMCs are not terminally differentiated and demonstrate remarkable plasticity in response to varied environmental cues, switching between contractile and synthetic (proliferative/migratory) phenotypes as needed for vascular development during embryogenesis, and, for remodeling and repair in adulthood [85]. Dedifferentiation to a synthetic phenotype is marked by decreased (sometimes undetectable) expression of SMC-selective genes that encode components of the contractile apparatus (e.g., α-SMC, SM-MHC, SM22α) [60]. To complicate matters further, many of these contractile markers are also expressed transiently in other cell types during repair and in disease [86]. For instance, macrophages have been reported to express α-SMC and SM22α in response to TGF-β [87] and thrombin [88], while cholesterol loading of SMC results in the induction of several markers typically associated with macrophage function related to phagocytosis and antigen presentation [89]. The relative contribution of resident and myeloid progenitors to the transformed cellular pool is also uncertain. Thus, unambiguous identification of cells of SMC origin in vascular lesions is very challenging. Unfortunately, previously employed lineage-tracing methods to positively identify SMC have lacked sufficient rigor in order to define the contribution of SMC to disease progression.
Importantly, beyond issues with the in situ identification of VSMC per se, technical difficulties are also encountered in definitively ascertaining the osteochondrocytic VSMC phenotype. The identification of this putative phenotype in culture and in vivo is principally based on detecting the de novo expression of pro-osteoblastic (Runx2, osterix, Msx) or pro-chondrocytic (Runx2, Sox9) transcription factors and their downstream gene products (e.g., OPN, OCN, MGP, TNAP, Col II). However, several of these so-called bone markers lack specificity as they are synthesized by cell types besides bone cells (e.g., macrophage, endothelial cells) and are therefore not truly specific for osteogenic transformation [58, 90]. Unfortunately, relatively few studies have confirmed expression of these markers at the level of both the protein and gene. Sole reliance on changes in gene expression can be misleading due to the oversensitivity of modern-day RT-PCR . Fewer studies still, demonstrate that an often small-magnitude change in transcription factor expression translates into meaningful increases in transcriptional activity. False positive signals are also frequent with immunohistochemical analysis due to the nonspecific binding of antibodies to mineral deposits and lipidic necrotic/debris often present in atherosclerotic plaques, but perhaps more critically due to the binding of circulating “bone” proteins (e.g., MGP, OPN, OCN) to mineral phases for which they purposefully have very high affinity. Local synthesis by transformed cells cannot therefore be established by positive protein staining alone.
3.1.3 Evidence for VSMC Osteochondrogenesis in Human Vessels
With respect to studies of non-uremic human vessels obtained at autopsy or at the time of surgery (e.g., endarterectomy), “bone” protein expression has been detected both in calcified intimal and medial lesions (Table 2). In particular, while generally absent in undiseased vessels, early analyses of atherosclerotic plaques showed fairly consistent expression (gene) and staining (protein) of OPN, with the latter mainly localized to intimal macrophages and VSMC surrounding mineral deposits [90, 91]. Although constitutively expressed by VSMC [92], MGP expression has also been spatially related to calcified foci but appears generally downregulated in affected vessels, especially in those with severe MAC [56, 61]. Osteogenic transcription factors (Runx2, Sox9) and their downstream gene products (TNAP) have also been detected in conjunction with increased Col II expression, suggestive of a chondrocytic-like transdifferentiation and activity of VSMC, and are consistent with other histological manifestations of cartilaginous metaplasia that have previously been described [79].
Table 2
Evidence for VSMC osteochondrogenesis in human vessels
Study | Cohort | Arterial site(s) | Sample size (n) | Bone protein (detection method) | Localization |
---|---|---|---|---|---|
Bostrom et al. [52] | NS | Carotid | 3 | BMP2 (ISH) | Intimal SMC |
Shanahan et al. [56] | NS | Coronary | 18 | OPN (ISH, IHC) | Intimal M, occ. medial M (no staining in normal vessels) |
MGP (ISH, IHC) | Intimal M, occ. medial M/SMC (low-level intimal and medial SMC staining in normal vessels) | ||||
Fitzpatrick et al. [224] | NS | Coronary | 7 | OPN (IHC) | Intimal (no staining in normal vessels) |
Giachelli et al. [225] | NS | Carotid, coronary | 11 | OPN (IHC) | Intimal M/SMC & mineral staining (occ. intimal staining in normal vessels) |
O’Brien et al. [90] | NS | Coronary | 19 | OPN (ISH, IHC) | Intimal M/SMC & mineral staining (no staining in normal vessels) |
Shanahan et al. [56] | Diabetic | Tibial, popliteal, tibio/peroneal, femoral | 9 (severe MAC) | MGP (ISH, IHC) | Highly expressed in SMC adjacent to mineral (medial SMC staining in normal vessels) |
OPN (ISH, IHC) | Intimal M, medial mineral staining (no staining in normal vessels) | ||||
ALK, OCN, Col II (RT-PCR) | Medial (undetectable in normal vessels) | ||||
Medial OPN BMP2, SM22a, Col I expression similar to normal vessels | |||||
Tyson et al. [61] | NS | Aorta, carotid | 40 | Runx2 (RT-PCR) ALK, OCN (RT-PCR, ISH) | Intimal SMC & M, medial SMC; Msx2 intimal SMC |
Runx2, Sox9 undetectable by ISH; highly variable expression Sox9, MGP, Col I, Col II | |||||
MGP expression in noncalcified vessels; MGP downregulated in some calcified samples | |||||
Bobryshev [219] | NS | Aorta, carotid | 28 | Col II, Sox9 (IHC) | Highly variable intimal SMC expression |
Neven et al. [79] | Transplant donors | Aorta | 7 (MAC in 4) | Sox9, Runx2, Col II (IHC) | Medial staining in calcified vessels (no staining in normal vessels) |
CKD | |||||
Moe et al. [226] | ESRD (predialysis/HD) | Epigastric | 39 (no MAC in 27) | OPN, ALK, OPN, Col I (IHC) | Medial staining correlated with calcification severity |
75 % vessels without MAC had staining for 1+ bone protein | |||||
Gross et al. [96] | Renal/non-renal | Coronary | 46 | OCN (IHC) | Intimal staining higher in renal vs. non-renal; OPN similar staining |
Shroff et al. [39] | Pediatric predialysis/dialysis | Omental, epigastric, mesenteric | 34 (MAC in 6 HD) | Runx2, Osx, ALK (IHC) | Medial staining in dialysis vessels; cytoplasmic transcription factor staining |
Minimal staining in normal/predialysis vessels | |||||
Yoshida et al. [93] | CKD 2–3/HD | Coronary | 19 (mostly IAC) | OPN, Runx2 (IHC) | CKD 2–3: intimal (occ. medial) OPN, no Runx2; HD: intimal/medial OPN/Runx2 |
O’Neill et al. [94] | ESRD (predialysis/HD) | Breast | 19 (MAC in 18) | OCN, Runx2, Osx, Sox9 (IHC) | No cellular staining, minimal medial OCN staining associated with mineral |
What then is the evidence for VSMC-mediated VC in human CKD ? Currently, data is limited to a small number of studies of medium-sized muscular arteries (epigastric, omental, mammary) acquired at transplantation and catheter insertion or of coronary vessels obtained at autopsy. In landmark studies by Shroff and colleagues, it was shown that calcium-containing deposits accumulate in the arterial wall of predialysis and dialysis vessels compared to mesenteric vessels obtained from healthy controls during elective surgery. However, in the case of predialysis vessels this deposition was associated with preserved vessel architecture, normal inhibitor concentrations, no evidence of osteochondrocytic transdifferentiation (Runx2 expression, ALP activity), MV release, and a normal number of VSMC [55]. Indeed, only in vessels from dialysis patients was there convincing evidence of VSMC loss (partly due to apoptosis—although the absolute numerical contribution was small), Runx2 staining, an increase in ALP activity, reduced levels of inhibitors (fetuin-A and MGP), and release of mineral-laden MV. Moreover, although ex vivo experiments on isolated intact vessel rings showed increased time-dependent calcium accumulation in predialysis vessels compared to controls (although of much lesser magnitude compared to the increase in dialysis vessels), there was no significant change in osteochondrocytic markers or cell viability, even in high calcium/phosphate medium (2.7 mM Ca, 2.0 mM P). Taken together these data suggest that factors specific to the dialytic milieu drive accelerated VSMC-mediated calcification in patients with ESRD, and, that other, possibly noncellular (passive), mechanisms of mineralization may predominate prior to the initiation of dialysis therapy. Critically, however, these were vessels from pediatric patients and appeared free from significant atherosclerotic disease (no intimal thickening or plaques were observed). This may be germane, as concurrent atherosclerotic disease and impairment of endothelial function, more typical of vascular disease in adult CKD, in addition to greater cumulative exposure to inappropriate environmental cues, may induce cell-mediated mineralization pathways at an earlier stage in adult CKD progression. However, arguing against this possibility, studies of coronary vessels obtained at autopsy by Yoshida et al. showed minimal intimal OPN staining (no medial staining) and absent Runx2 staining in adults with stages 2–3 CKD, with only variable intimal and medial staining present in sections from hemodialysis patients [93]. Moreover, a recent study of exclusively medial calcification in breast arteries from patients with ESRD showed no immunohistological evidence of VSMC osteochondrocytic transdifferentiation (OCN, Runx2, osterix, Sox9) or cell loss due to apoptosis in any section analyzed [94].
Based on the available evidence and aforementioned technical limitations and deficiencies, it is difficult to conclude that there is a major role for medial VSMC-mediated arterial mineralization in patients with CKD prior to the initiation of hemodialysis therapy. Even then, the occurrence would appear sporadic and inconsistent. Indeed, despite universal exposure to the pro-calcific uremic milieu only a proportion of patients receiving chronic hemodialysis have significant MAC by imaging or histology [95]. An explanation for this variable disease penetrance is not currently forthcoming. Yet it should also be stressed that with the exception of two studies on coronary vessels [93, 96], the analyses conducted to date have been on small-to-medium sized muscular arteries that are generally free from intimal/atherosclerotic disease involvement and which may therefore not be representative of changes occurring in some larger elastic vessels (e.g., aorta). This may in part also explain the apparent disparity with findings in some animal models of uremia, where studies have mainly centered on changes in VSMC phenotype in the aorta and where phenotypic switching would appear to occur relatively early in disease progression [97–99]. On the other hand, major differences in the pathogenesis of vascular disease in man and rodents are not without precedent and, specifically with respect to VC, there are examples of marked differences in phenotype resulting from mutations in key regulators of mineralization. For instance, MGP−/− mice have a very strong phenotype of massive medial arterial calcification that leads to vessel rupture, thrombosis, and death within several months of birth. In humans, however, inactivating mutations in MGP (Keutel syndrome) manifest with diffuse cartilage calcification, short distal phalanges, and pulmonary disease, but otherwise a very mild cardiovascular phenotype in which arterial calcification is surprisingly infrequent [100]. Conversely, inactivating mutations in ENNP1, encoding the pyrophosphate synthesizing ectoenzyme nucleotide pyrophosphatase/phosphodiesterase, results in the devastating (but variably penetrant) syndrome, generalized arterial calcification of infancy (GACI), which is characterized by severe systemic MAC that develops in utero, leading to widespread neointimal expansion and vessel occlusion, multiorgan failure, and non-atherosclerotic-associated myocardial infarction within a few months of birth [101]. Ablation of the homologous gene, Npps, in mice, however, results in ossification of the spinal ligaments and periarticular calcification but with relatively minor arterial involvement and only a modestly shortened lifespan [102, 103].
3.1.4 On the Primacy of Changes in VSMC Phenotype to Vascular Calcification
Notwithstanding these issues, even if VSMC osteochondrocytic transformation does occur in vivo, it is not certain whether such changes in phenotype are a primary initiating event driving subsequent calcification or an adaptive secondary response to increased cellular mineral exposure. A similar argument can be made for the synthesis of osteopontin by macrophage resident in calcifying lesions in fetuin-A −/− and MGP−/− mice [104]. From a teleological perspective the latter appears logical as it may represent an attempt by the cell to efficiently organize and immobilize mineral in a less toxic form outside of the cell, and in so doing, limit the spread of potentially noxious nanocrystalline particles. Although several studies in MGP−/− [83] and high-phosphate-fed uremic mice [98] suggest that VSMC transformation may precede arterial calcification, mineral deposition was only assessed by histochemical staining in these studies and not by actual measurement of calcium content, which may not be sufficiently sensitive to detect early microcalcifications. Furthermore, other studies in identical models have failed to replicate such findings [105], and such a temporal relationship has yet to be unequivocally demonstrated in either animals or man. These mechanistic considerations may hold important therapeutic implications, as targeting VSMC transdifferentiation processes prematurely may disrupt appropriate adaptive responses and be harmful.
3.2 Vascular Smooth Muscle Cell Matrix Vesicle Release
Ghadially and colleagues first described finding electron-dense granular and membranous bodies in the matrix of human rabbit articular cartilage in 1965 [106]. Originally termed “matrical lipidic debris,” these authors and others considered such particles to be “extruded cellular processes” of chondrocytes and by-products of normal cell remodeling and turnover [106, 107]. At some sites (e.g., the calcifying zone of cartilage) this material was seen to calcify. Subsequently, detailed EM studies by Anderson and Bonucci suggested that these extracellular double membrane-bound vesicles, termed matrix vesicles (MV), were closely associated with the very early stages of bone and cartilage mineralization [108, 109]. MV represent one of several types of crudely classified extracellular vesicle that have been isolated from the “cell dust,” which in addition to MV encompasses vesicular inclusions such as exosomes, ectosomes/microparticles, and apoptotic bodies that differ in size, density, morphology , and lipid/protein composition and apparent function, although there appears to be a considerable overlap. As defined, MV are a heterogeneous population of typically ovoid-shaped membrane-bound extracellular bodies (30–300 nm) released from the plasma membrane of cells involved in physiological mineralization such as osteoblasts, chondrocytes, and odontoblasts [110, 111]. Depending on their cargo, MV are capable of nucleating hydroxyapatite crystals on their exterior and interior surfaces. Mineralization-competent MV appear enriched for annexins [2, 5, 6] and acidic phospholipids (e.g., phosphatidylserine) which facilitate calcium binding, ion influx into the vesicle lumen, and formation of the nucleation core [112]. In osteoblast and chondrocyte-derived MV, alkaline phosphate has also been variously detected, which, by unknown mechanisms, is thought to drive the generation and uptake of phosphate from PPi [113]. In the absence of inhibitors (e.g., fetuin-A and MGP), intravesicular mineral accrual drives the formation and expansion of initially amorphous calcium phosphate particles before undergoing ripening to form crystalline hydroxyapatite [63, 114]. On release from the MV membrane (also by unknown mechanisms) and exposure to the extracellular milieu, these crystals may form a nidus or template for further mineral crystal formation via homologous nucleation [115]. MV appear to bind avidly to certain ECM components such as glycosaminoglycans and collagen, with MV-associated mineral crystals consequently seeding these structures in situ [116].
The existence of such specific “organelles of calcification” continues to be questioned by some authors, who cite the fact that mineral-containing vesicles indistinguishable from MV are also frequently present in noncalcifying regions of articular cartilage and noncalcifying elastic cartilages and, further, that a distinct and uniform population of vesicles of constant morphology and size has not been observed. Indeed, lipidic debris from dead and dying cells has long been known to serve as a nidus for mineralization. Isolated vesicles are also reportedly unable to actively transport calcium [117]. Despite these often vociferous protestations [118], MV are now widely regarded as having an important role in the initiation of skeletal mineralization [110] and likely represent at least one plausible pathway by which mineral is delivered to the mineralization front in calcifying tissues.
Emerging evidence also suggests a role for calcifying MV in early pathological mineralization. MV have been observed in atherosclerotic plaques [119], MAC [114], as well as in calcifying aortic value disease [120], where they may initiate mineralization in a manner similar to that proposed for bone and the dentition. In vitro studies show that high extracellular calcium concentrations induce the release of mineral-loaded MV from VSMC [63], while macrophages have also recently been shown to release MV in response to mineral and inflammatory stressors like TNF-α [119].
Elevated calcium levels can also induce apoptotic death of VSMC leading to the extrusion of intracellular calcium stores, further augmenting local tissue concentrations, and the release of mineral crystal-nucleating apoptotic bodies [57, 63]. Calcium also plays a role in triggering MMP2-mediated degradation of elastin and in the exposure of annexin 6-phosphatidylserine nucleation complexes [63, 121]. Perhaps more importantly, chronic exposure to high calcium levels and the resultant cell attrition/mineral deposition is postulated to deplete the vascular tissues from essential calcification inhibitors [63, 114]. Thus the high calcium and phosphate concentrations frequently encountered in patients with advanced CKD (assumed to reflect a net positive total body balance of calcium and phosphate) appear to act synergistically, driving disparate processes that together lead to cell-mediated mineralization of the vasculature [122].
4 Biomineralization: Loss of Inhibition
Beyond these cell-mediated mechanisms, it is instructive to consider the physicochemical pathways driving all mineralization processes that are harnessed biologically to create ordered deposition in the body. Crystallization, the transition of matter from the solvated phase into a crystal lattice, is a complex physiochemical process that is governed by various thermodynamic and kinetic considerations [123]. These relate to the degree of solute supersaturation relative to a given solid phase, which in turn is dependent on the composition and size of the initial solid-phase nuclei, the presence of active heterogeneous nucleators or templates that facilitate nucleation, and the presence (or absence) of crystal growth inhibitors/modifiers [124]. All these factors impinge on the molecular “energy landscape,” which determines the rate and pathway of transformation of a metastable, high free energy solution phase to a lower free energy crystalline phase.
Hydroxyapatite is the most stable calcium phosphate phase (having a lower free energy compared to ionic and precursor states) and its formation although kinetically unfavorable is strongly thermodynamically favored [124]. Thus, over time, hydroxyapatite will eventually seed from fluids containing physiological concentrations of calcium and phosphate, if crystal nucleators are present. Consequently, multiple local and systemic inhibitory systems have evolved to regulate mineralization and restrict hydroxyapatite nucleation, growth, and deposition to physiological sites (bone, cartilage growth plate, dentin, and cementum), as well as cargo or “chaperone” systems to facilitate clearance of mineral nuclei from extraosseous locales. Indeed, it is worth noting that Gersh and McLean demonstrated more than 70 years ago that the loading of plasma with supraphysiological amounts of calcium and phosphate does not result in spontaneous precipitation of mineral in the circulation due to the formation of soluble colloidal protein-bound complexes of calcium phosphate that are cleared rapidly by the reticuloendothelial system [125–127].
The importance of these inhibitory networks is strongly reinforced by the fact that genetic ablation of protein inhibitors of mineral crystal nucleation (e.g., matrix Gla protein, MGP) or disruption of enzyme systems that generate potent biochemical inhibitors of mineral crystal growth (e.g., pyrophosphate, PPi) in mice and in humans (see below) can result in a phenotype of spontaneous severe calcification of the vasculature and other soft tissues [82, 128, 129]. Critically, this occurs in the absence of disturbances in systemic mineral biochemistry (i.e., normal serum calcium and phosphate concentrations). Indeed, the spatial restriction of mineral deposition to physiological sites of mineralization appears to relate to the co-expression of (1) enzyme systems that degrade endogenous inhibitors of mineral crystal growth (e.g., TNAP, tissue nonspecific alkaline phosphatase); (2) an active self-assembling macromolecular scaffold (e.g., type I collagen) that provides the necessary spatial constraints and local mineral supersaturation for crystal formation; and (3) noncollagenous acidic phosphoproteins (e.g., dentin matrix protein 1, DMP-1; matrix Gla protein, MGP) and phospholipids that can direct mineral crystal nucleation, aggregation, and the hierarchical assembly of oriented crystalline hydroxyapatite platelets within the organic matrix [130, 131]. Such co-expression appears unique to terminally differentiated mineralization-competent osteoblasts, odontoblasts, and chondrocytes [132].
These seminal works have led to the “loss-of-inhibition” model of mineralization whereby deposition of mineral only occurs when these inhibitory systems are downregulated, either physiologically during skeletal ossification or pathologically leading to ectopic tissue calcification.
The corollary of these findings is that, for ectopic calcification to occur, endogenous inhibitory systems must be overwhelmed or compromised and a nidus for mineral crystal deposition must be present (e.g., cell debris, apoptotic bodies, damaged/fragmented elastin fibers, matrix vesicles). Although elevations in calcium or phosphate may clearly promote ectopic calcification (by virtue of increased supersaturation), they are neither required nor alone sufficient to induce ectopic mineralization at concentrations observed in vivo.
4.1 Local and Systemic Inhibitory Networks
VSMCs are normally highly resistant to mineralization and only when inhibitory mechanisms become compromised does calcification occur. Amongst the best characterized inhibitors of calcification are fetuin-A , a systemically acting glycoprotein secreted by the liver [84], and MGP and PPi, both produced locally by osteoblasts and VSMC [82, 133]. Reynolds et al. demonstrated that fetuin-A is internalized by synthetic VSMC exposed to high calcium and phosphate concentrations, wherein it regulates several of the key steps that lead to VSMC calcification: blocking caspase-mediated apoptotic signaling cascades; promoting phagocytosis of calcifying cells and apoptotic bodies; and loading into matrix vesicles, preventing mineral crystal growth [114, 134]. Interestingly, fetuin-A also appears to be loaded into matrix vesicles elaborated from chondrocytes during physiologic chondrogenesis [135]. Ablation of the fetuin-A gene results in a phenotype of extensive soft-tissue calcification and altered bone growth [136]. Like fetuin-A, MGP is also loaded into matrix vesicles wherein it inhibits mineral crystal nucleation [63, 114]. Additionally, MGP serves as soluble decoy receptor for osteoinductive BMP2 [137, 138], a potent activator of SMAD (Mothers Against Decapentaplegic homolog proteins)-dependent Runx2, Msx2, and p21 signaling cascades, as well as other members of the TGF-β superfamily. Critically, MGP activity requires vitamin K-dependent γ-carboxylation [139]; thus nutritional vitamin K deficiency or inhibition of active vitamin recycling with warfarin is associated with impaired functionality of this protein [140]. MGP knockout mice develop severe vascular calcification rapidly after birth [82]. PPi, on the other hand, is a crystal poison, directly binding to mineral crystals blocking further growth [141]. PPi is degraded by tissue nonspecific alkaline phosphatase (TNAP) [142], which appears consistently upregulated during early VSMC mineralization in a number of in vitro and in vivo calcification models [142–145]. PPi is synthesized via ATP-dependent pathways catalyzed by ENPP1 [146]. As described above, inactivating mutations in ENNP1 result in the devastating phenotype of idiopathic infantile arterial calcification or GACI [101].
5 Mineral Nanocrystals: True Mediators of Mineral Stress?
The elevation in plasma phosphate concentrations often seen in patients with advanced CKD (eGFR <30 ml/min/1.73 m2) [147, 148] is widely considered to be the principal driver of accelerated vascular calcification in this setting [122, 149–153]. However, while there is in vitro evidence that phosphate may be directly damaging to certain cell types (e.g., EC, VSMC) at levels equivalent to plasma concentrations found in some ESRD patients (>2 mmol/L) [65, 154], emerging data suggests that the toxic effects of phosphate may be mediated by calcium phosphate nanocrystals rather than soluble ionic phosphate itself [68, 141, 155–157].
Standard culture media are relatively simple buffered salt solutions without the complex biochemistry and colligative properties of serum. Importantly, many culture media contain much higher ionized Ca2+ concentrations than found in human serum (e.g., DMEM ~1.8 mmol/L vs. 1.2 mmol/L) and, although supplementation with FBS does supply some calcium-binding/mineral crystal inhibitors (e.g., fetuin-A ), mineral precipitation is strongly kinetically favored compared to precipitation in human serum. Thus, even relatively modest elevations in calcium or phosphate may lead to spontaneous mineral nanocrystal formation in culture media.
This was recently highlighted by Sage et al. who found that mineral nanocrystals were formed within 72 h in cell-free culture medium (10 % FCS-DMEM) containing 2 mmol/L phosphate (and 1.8 mmol/L free calcium) incubated at 37 °C [68]. Nanocrystal formation was abrogated by the addition of PPi, a crystal poison, and grossly augmented by the omission of FCS from the medium, indicating that components in serum have a marked effect on nanocrystal formation [68].
Nanocrystals of basic calcium phosphate (BCP) consisting of hydroxyapatite or octacalcium phosphate have been reported to strongly stimulate a number of cell types, in vitro [68, 156]. The rise in intracellular calcium concentration that accompanies lysosomal dissolution of internalized BCP crystals, or calcium influx following direct or receptor-mediated crystal-cell interactions, induces the synthesis of various intracellular and secreted mediators, alters membrane permeability and reduces cell viability. Of particular importance, BCP crystals have been shown to induce the production of osteogenic proteins by VSMC (e.g., BMP2 and osteopontin), independent of Runx2, as well as inducing cell death at high levels without any increase in culture phosphate concentration [68, 155]. Critically, exposure of VSMC to high phosphate medium (2 mmol/L) after removal of nanocrystals by centrifugation does not appear to generate the same osteogenic response [68]. Crystal nucleation inhibitors, PPi and phosphonoformic acid, also reportedly block high phosphate-induced VSMC mineralization [156]. Interestingly, there is evidence that calcium phosphate nanocrystals may regulate the osteogenic response in osteoblasts. Khoshniat et al. reported that cell-crystal interactions mediated by caveolae-containing lipid rafts led to early response kinase (ERK) 1/2-stimulated mineralization-associated gene expression [158]. Signaling via this pathway was abolished by inhibition of crystal formation with phosphocitrate, despite high phosphate/calcium culture conditions [158].
BCP-induced apoptosis of VSMC may lead to atherosclerotic plaque destabilization and rupture, while in the medial layer, enhanced VSMC mineralization may potentiate the loss of large vessel compliance [23]. In the macrophage, BCP crystals induce secretion of tumor necrosis factor (TNF)-α, interleukin (IL)-1β, and IL-18, via Toll-like receptor 4, protein kinase C-α/mitogen-activated protein kinase and nucleotide-binding domain, leucine-rich-repeat-containing family pyrin domain-containing 3 (NLRP3) inflammasome-dependent pathways [159, 160]. The inflammasomes are cytosolic multicomponent proteolytic complexes, which once primed, activate caspase 1-mediated processing of pre-formed pro-IL1β and pro-IL-18 cytokines to their mature secreted forms [161]. Considerable interest now surrounds the role of the NLRP3 inflammasome activation in response to a diverse array of metabolic damage-associated signals (DAMPs) and its role in various chronic metabolic disorders (e.g., atherosclerosis, type 2 diabetes, obesity, and the gouty arthritis) [162]. In particular, NLRP3 priming and activation appears to be central to the proinflammatory response to crystalline and aggregated particulate matter (e.g., cholesterol, MSU and BCP crystals, and islet amyloid polypeptide). BCP-induced inflammation contributes to the cartilage deterioration, subchondral remodeling, and synovitis seen in severe osteoarthritis and other destructive arthropathies [163]. Indeed intra-articular deposition of BCP crystals is strongly associated with the severity of cartilage degeneration [164]. In fibroblasts , on the other hand, exposure to BCP results in matrix metalloproteinase production and a proliferative response [165, 166]. Consequently, BCP crystals may play an important, but cell-specific, role in the genesis of a number of pathologies.
Crystal size appears to be a key determinant of proinflammatory and apoptotic potency [167]. Crystals isolated from aortic and carotid plaques range in size from 50 nm to 8 μm [155, 168]; however, studies in both the macrophage and VSMC suggest that only crystals less than 1-2 μm in diameter are strongly stimulatory [155, 169]. Interestingly, in studies of synthetic hydroxyapatite crystals released from prosthetic implants, Laquerriere et al. reported that needle-shaped particles were most potently inflammatory [170]; thus crystal shape may also be important. Indeed, phagolysosomal overloading with crystalline particles of acute-angle geometries, as seen with silica dust, cholesterol crystals, asbestos, and carbon nanotubes, may result in phagolysosomal rupture and subseuqent activation of the NRLP3 inflammasome [171, 172].
Agglomeration of mineral nanocrystals has also been reported to induce ROS generation and proinflammatory cascades and affect membrane integrity and cell viability of human and murine macrophages in vitro [173]. The pathophysiological significance of nanoparticulate agglomerates is uncertain, however, as these effects have only been observed in serum-free or low serum media. Indeed, there is evidence that serum components may stabilize smaller particles and reduce agglomeration potential [173].
In aggregate, it is likely that both “passive” and “active” components contribute to vascular mineralization, each with potentially greater or lesser importance, depending on the vessel or pathological context. Moreover, the distinction between these elements appears overly simplistic. For instance, matrix components (e.g., elastin, MGP, PPi) not only are synthesized by cells but also have independent effects on cell function and phenotype, distinct from their effects on mineral crystal nucleation and growth [174, 175]. Thus, it would be more accurate to consider VC as a hybrid of interacting biochemical and cellular processes. However, what is far less clear is whether changes in VSMC phenotype (osteochondrocytic transdifferentiation ) or viability represents the initial event driving subsequent VC, as supported by some studies [83, 98], or, as suggested by others [157], whether such cellular transformations and attrition are mainly a secondary adaptive/maladaptive response to mineral deposition in the surrounding matrix that results from compromised inhibitory mechanisms and the thermodynamic tendency for calcium and phosphate to spontaneously precipitate.
6 Inflammation and Vascular Calcification: Cause and Effect?
Aging is associated with the development of a chronic low-grade proinflammatory phenotype, referred to as “inflammaging” [176]. This is characterized by amplified inflammatory cytokine production and dysregulation of the immune system, which results in increased susceptibility to infection but also has important effects on the function of other tissues like the vasculature [177]. Multiple lines of evidence suggest that proinflammatory cascades are amplified in CKD . Indeed, age-associated central arterial stiffening has been independently associated with serum C-reactive protein (CRP) levels and other proinflammatory cytokines in patients with non-dialysis-dependent and dialysis-dependent CKD, as well as in the non-CKD elderly population [178, 179]. Interestingly, there is also strong evidence of increased arterial stiffness in patients with chronic inflammatory conditions such as inflammatory bowel disease, rheumatoid arthritis, and systemic lupus erythematosus, who are also at higher CV risk [180–184]. In this setting, treatment with anti-inflammatory agents (e.g., TNFα blockade) is associated with reduced arterial stiffness and inflammation (assessed by 18F-fluorodeoxyglucose (FDG)-PET) [183, 185]. Importantly, these chronic inflammatory disorders are also associated with increased prevalence and severity of vascular calcification [186–188], and variously with lower levels of systemic mineralization inhibitors such as fetuin-A [189, 190].
Like many chronic diseases, CKD is considered a proinflammatory state with increased circulating concentrations of cytokines such as TNFα, and in particular, IL-6 [191], a key marker of the senescence-associated secretory phenotype. Indeed, in hemodialysis patients, serum IL-6 levels are strongly correlated with oxidative stress, telomere attrition, and all-cause mortality [192]. Elevated serum C-reactive protein levels have been associated with reduced telomere length in mononuclear cells from hemodialysis patients [193]. Moreover, changes in the stem cell pool usually observed with aging have also been noted in younger uremic patients [194], typified by the depletion of endothelial progenitors (CD34+), bone marrow-derived stromal cells, and expansion of the proinflammatory and pro-atherogenic CD14+/CD16+ monocytic pool [195, 196]. Together these findings implicate accelerated age-related changes in immune cell function, as an important component of the premature aging phenotype seen in CKD [4]. Indeed, cells of the immune system are subject to enormous proliferative demand, especially in advanced CKD, and are therefore particularly sensitive to telomere attrition and telomere-initiated senescence [197]. Furthermore, the secretory products of these senescent cells (e.g., BMP2, IL-6) may be critical in reinforcing proinflammatory, osteogenic and growth arrest signaling in the vasculature [198].
In support of the direct effect of proinflammatory cytokines on calcification propensity, in vitro studies have demonstrated the ability of these factors to induce osteochondrocytic differentiation and resultant mineralization of VSMC through convergent intracellular signaling pathways. TNFα, mainly released by activated macrophages but also variably by VSMC, upregulates expression of osteoblast specific transcription factor (Osf2) and enhances expression and activity of ALP via cAMP-dependent pathways [199]. TNFα also induces VSMC apoptosis via cAMP-mediated inhibition of AMP-activated protein kinase (AMPK)-dependent Gas6/Akt signaling and increases Msx2 expression via the nuclear factor κ-light chain enhancer of activated B-cells (NF-κB) pathway, also leading to enhanced ALP activity [200]. Systemic and locally synthesized IL-6 can also enhance ALP activity and promote osteochondrocytic differentiation via activation of Janus kinases and signal transducers and activators of transcription (STAT) signaling cascades [201]. Furthermore, Awan et al. recently reported that administration of a monoclonal antibody against Il-1β attenuated the development of aortic calcifications in high-fat-fed adult LDLR−/− mice compared to placebo [202].
Elegant molecular imaging studies have provided compelling evidence of the close temporal and spatial relationship of inflammatory and osteogenic vascular processes in vivo. Using intravital microscopy and sensitive fluorogenic probes, Aikawa et al. were able to demonstrate the co-localization and increasing intensity of mineralization (bisphosphonate-conjugated agent) and inflammatory activity/macrophage burden (NIRF-conjugated iron nanoparticles) in intimal plaques of carotid arteries isolated from ApoE−/− mice [203]. The same group subsequently extended these findings in uremic ApoE−/− mice, but also showed a reciprocal relationship between bone mineral density (osteoporosis), inflammatory activity, and mineral accrual within vascular and valvular lesions [204]. Bone mineral loss in uremic mice appeared accelerated compared to non-uremic animals. More recently, Abdelbaky et al. have reported on the longitudinal association between focal inflammation determined by 18F-FDG uptake and calcification in the thoracic aorta in patients with serial PET/CT analyses [205]. Here, these authors showed evidence that FDG uptake preceded calcium deposition at the same arterial sites, emphasizing the primacy of inflammatory changes in driving subsequent mineralization of atherosclerotic plaques.
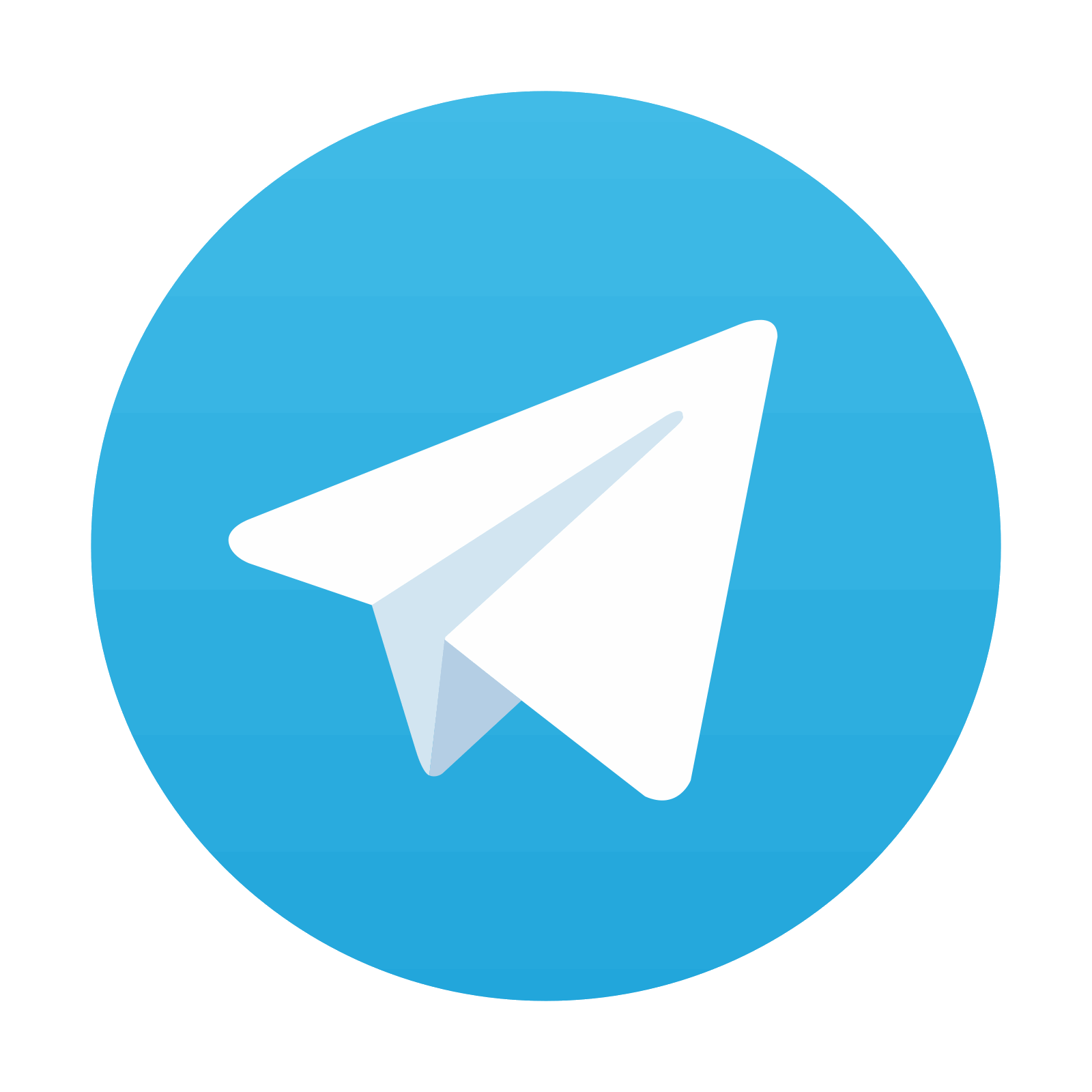
Stay updated, free articles. Join our Telegram channel
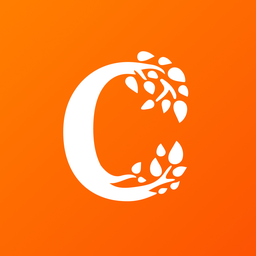
Full access? Get Clinical Tree
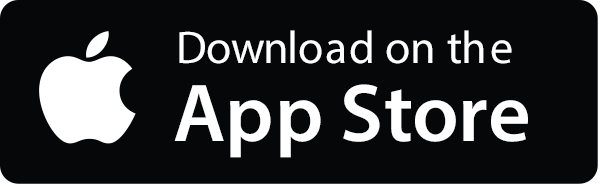
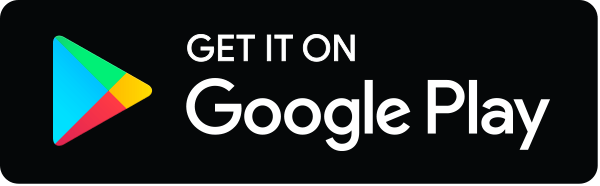