Fig. 6.1
An acetylation reaction, catalyzed by the enzyme aac (6′)-Ib-cr, of the (=NH) moiety within the piperazine ring of ciprofloxacin. Levofloxacin (bottom) possesses a methylated piperazine residue (indicated by the arrow), virtually protecting the molecule from N-acetylation reactions
Although the levels of resistance conferred by AAC are modest, the combined effect of AAC and the major resistance determinant qnrA can generate high levels of resistance to ciprofloxacin (MIC: 0.008 mg/L [WT E. coli]; 0.25 mg/L [only qnr in E. coli], 1 mg/L [qnr plus aac (6′)-Ib-cr in E. coli]) [51].
Of equal importance is the effect exerted by AAC on the fluoroquinolone mutant prevention concentration (MPC) (up to 16-fold increase: 0.2 → 3.2 mg/L), which facilitates the survival of target site mutants [10, 51].
As described above, the acetyltransferase encoded by the gene aac (6′)-Ib-cr induces resistance to fluoroquinolones through the modification of the = NH piperazine residue of drugs like ciprofloxacin or norfloxacin. Interestingly, the FQ levofloxacin has a methylated piperazine residue (Fig. 6.1), which is virtually protected from the action of AAC. This cannot only prevent the emergence of resistance in pathogens carrying the aac (6′)-Ib-cr gene, but does not allow the combined effect of aac (6′)-Ib-cr and QnrA, nor the increase of the MPC in exposed pathogens. Thus, where resistance rates caused by the AAC are present or suspected (e.g., in case of an antibiogram reporting a gram-negative pathogen intermediate resistant to ciprofloxacin but not to levofloxacin), levofloxacin, or other “protected” FQs like prulifloxacin or pefloxacin, may be considered [8, 20, 21].
In the case of fluoroquinolone resistance in non-multidrug-resistant strains, or in the presence of adverse reactions to FQs (e.g., tendonitis, phototoxicity, ECG-QT interval elongation), or in patients at high risk for these reactions, alternative agents like trimethoprim (or the co-trimoxazole combination) can be administered orally for a period of 12 weeks after the initial diagnosis. Notably, co-trimoxazole should be administered only in areas where resistance rates for E. coli/Enterobacteriaceae are inferior to 20 %. The patient should then be reassessed and therapy only continued if pretreatment cultures are still positive and the patient has reported positive effects from the treatment (EAU guidelines: http://uroweb.org/wp-content/uploads/19-Urological-infections_LR.pdf). Other drugs that may be considered in these cases are tetracyclines (e.g., doxycycline) and macrolides (e.g., azithromycin), though none of these agents is active against P. aeruginosa. Notably, administration of macrolides, alone or in combination with other antibacterials [37], may represent a convenient therapeutic option due to the optimal prostatic penetration of these agents; their activity against S. aureus, C. trachomatis, and anaerobes; their marked anti-biofilm activity; and their interesting immunomodulatory capacity. However, it should be emphasized that Enterococci are not sensitive to macrolides, and gram-negative pathogens show variable response to these agents.
Trimethoprim, tetracyclines, and macrolides have a good safety profile. However, certain macrolides can elongate the QT interval of the electrocardiogram [23] and should not be administered to patients at risk for polymorphic ventricular tachycardias.
In case of prostatic infections involving anaerobic protozoa like Trichomonas vaginalis or certain FQ-resistant anaerobic bacteria, a therapy with 500-mg metronidazole twice daily may be attempted, though this suggestion is only based on reports of single successful cases [1].
Like any therapeutic agent, antibacterials are subject to the laws of pharmacokinetics, describing the processes of drug absorption, blood-borne tissue distribution, metabolic transformation, and excretion.
Absorption of fluoroquinolones is generally optimal. The oral bioavailability (i.e., the fraction F of an oral dose that can actually reach the systemic circulation) of FQs is very high and most agents in this family show F levels of 90 % or higher. Other drugs used to treat chronic forms of bacterial prostatitis, like trimethoprim and doxycycline, also show excellent oral bioavailability (F > 95 %), whereas macrolides like azithromycin or clarithromycin show lower and variable absorption and bioavailability, ranging between 30 and 50 %.
Renal excretion of the unmodified drug (or of its active metabolites) is an essential characteristic of antibacterials that must be administered for treatment of urological infections like pyelonephritis or cystitis. This feature is not strictly required from drugs used to treat acute or chronic prostatitis, as these agents are distributed to the infected ducts of the gland via the prostate vasculature. Distribution of antibacterial agents to the ductal sites of infection in the prostate is indeed a critical issue, often affecting the success of treatment and greatly restricting the available therapeutic armamentarium. In order to reach infected ducts, a drug must cross the membranes of capillary endothelial cells, thus reaching the intracellular spaces and fluids of the gland parenchyma. Noncellular stromal components, the gland basement membrane, and different cell types (smooth-muscle cells, fibroblasts, etc.) may represent additional elements impeding the diffusion of antibacterial agents toward infected prostatic ducts (Fig. 6.2). However, the most critical obstacle to such diffusion process is represented by the presence of a putative “blood-prostate barrier.”


Fig. 6.2
Schematic representation of the diffusion route of an antibacterial agent (in this example: ciprofloxacin) from capillary vessels to pathogens colonizing a prostate ductal or luminal region. BC basal cell, BM basement membrane, CAP capillary vessel, FB fibroblast, GE glandular epithelium, LU lumen of gland or duct, SM smooth-muscle cell, ST noncellular stromal matrix components, UP infecting uropathogens. The dashed red line represents the putative barrier activity of tight junctions in the upper pole of columnar luminal cells; the green arrow shows the direction of the diffusion/distribution route
6.2.2 A Blood-Prostate Barrier?
Successful antibacterial therapy leading ultimately to the cure of CBP has always been hindered by the fact that a large number of commonly used antibiotics, including almost all penicillins and cephalosporins, have proven, with very few exceptions [12] to be ineffective against sensitive uropathogens colonizing prostate glands and ducts. This evidence represents the functional and empirical demonstration of the presence of a “barrier activity” or “fence activity” within the prostate gland, preventing the distribution of blood-borne antibacterial agents to the site of infection. This barrier/fence activity is commonly referred to as the “blood-prostate barrier.”
Barriers aimed at protecting gametes, embryos, and fetuses from external insults are well known and well described in humans as well as in animals. Blood-testis/blood-epididymis and ovarian blood-follicle barriers are typical examples of such structures. However, a comprehensive and systematic investigation aimed at demonstrating the presence and ultimate structure in humans of a blood-prostate barrier at the anatomical and ultrastructural levels has not been performed so far, to the authors’ knowledge.
Nevertheless, there is sufficient evidence at the molecular level to support the hypothesis that a “barrier activity” or “fence activity” may indeed impede the access of a number of antibacterial agents to the lumen of prostate acini or ducts. Such evidence emerged from studies aimed at demonstrating the loss of cell-to-cell connections during the neoplastic transformation of the prostate, enabling cells showing increasingly malignant phenotypes to detach from the primary mass, infiltrate the underlying tissues, and eventually metastasize. In particular, a number of studies focused on the major structural components of tight junctions. Tight junctions (TJs) are closely associated membranes of adjacent cells, joining together to form a barrier which is virtually impermeable to water and many solutes, including most drugs. For example, tight junctions connecting capillary endothelial cells in the central nervous system are the fundamental components of the blood-brain barrier.
Occludins, and the family of claudin proteins, are the major constituents of tight junctions. Bush and coworkers demonstrated that occludin is expressed in normal human prostate glands [7] and shows a characteristic occlusion staining pattern in the apical region of secretory luminal cells. Together with occludin, claudins are the most important components of TJs, and form the paracellular barrier controlling the flux of ions and small molecules through the intercellular spaces between epithelial cells. By immunofluorescence experiments, claudins 1, 3, 4, 5, 7, 8, and 10 were shown to be expressed and arranged in junctional patterns in the mouse prostate epithelium [52], and an effective barrier activity, completely blocking the passage of blood-borne radiolabeled dextran to ductal fluids, was functionally demonstrated in the rat ventral prostate [17]. In humans, claudins 1, 3, 4, 5, and 7 were shown to be expressed and organized in junctional patterns in the apical region of columnar luminal cells in normal, nonneoplastic prostatic epithelia both in prostate cancer patients and in subjects affected by benign prostatic hyperplasia [3, 55, 61] (Fig. 6.2).
The fact that most studies demonstrated junctional patterns of expression of claudins and occludin in the apex region of luminal cells of the prostate glandular epithelium does not restrict the role of “barrier” or “fence” to these cells alone. For example, El-Alfy and coworkers have shown that basal cells within prostate glands, present in an even proportion (~1:1) with luminal cells only in humans, show a strict intercellular continuity ensured by the presence of junction-like complexes, which may indeed further contribute to the “barrier effect” elicited by tight junctions in the apex of luminal cells [14]. In addition, claudin-1 was shown to be preferentially expressed in basal cells in normal human prostates, thus confirming the presence of junctional structures also in deeper, non-apical cellular layers of prostate glands [26].
In summary, molecular evidence supports the hypothesis that adjacent epithelial cells in the prostate gland may be tightly connected to exert a barrier activity, preventing blood-borne drugs to reach the glandular lumen and to target the sites of pathogen colonization and infection. Notably, the presence of tight junction components in the human prostate has been established enough to prompt investigation of claudins 3 and 4 as potential therapeutic targets of cytotoxic C. perfringens enterotoxin, for therapy of primary and metastatic prostate cancer [35]. Moreover, several authors have proposed the use of microbubble-enhanced ultrasound in order to disrupt tight junctions and the “barrier activity” preventing efficient delivery of antibacterials to the lumen of prostate glands. Interesting results have been obtained so far in animal models [34, 54].
To circumvent this barrier activity at the glandular level within the prostate, lipophilic antibacterial agents, able to cross lipid cellular membranes by simple diffusion, must be used in order to achieve drug concentrations sufficient to eradicate the causative pathogens at the site of infection and to prevent the emergence of chemoresistant bacterial strains. In this respect, it was shown that more lipophilic fluoroquinolones like moxifloxacin can achieve higher concentrations in prostatic secretions, compared to less lipophilic molecules like norfloxacin or ciprofloxacin [47, 64].
When chronic infection is caused by intracellular pathogens replicating within luminal and basal cells of the prostate gland (e.g., Chlamydia trachomatis, but also various facultative intracellular bacteria), a therapy with drugs unable to efficiently reach the gland lumen may be attempted, as these agents do not need to cross the junctional barrier located in the apical region of the secretory epithelium to exert their effect. In this respect, macrolides show excellent intracellular penetration and are very effective anti-chlamydial agents in patients affected by chronic prostatitis [36, 58].
6.2.3 Management of Resistant Infections in the Superbug Era: Which Options for Chronic Bacterial Prostatitis?
Global surveillance studies indicate that fluoroquinolone resistance rates are rising dramatically in almost all bacterial species [13]. In some parts of the world, FQ resistance is increasing in Enterobacteriaceae causing community-acquired or healthcare-associated urinary tract infections, as well as sexually transmitted conditions, and in Asia, resistance levels over 50 % are commonly found.
For example, resistance rates to antibacterials in Neisseria gonorrhoeae can be as high as ~100 %, particularly in Asia; similar to tuberculosis, gonorrhea is nowadays categorized as “multidrug resistant,” “extensively drug resistant,” and “untreatable” [62].
On this basis, guidelines are being adapted worldwide, and alternative protocols are being tentatively suggested, until new studies confirm these indications, or new agents are discovered [41].
In the worrisome era of “superbugs” [9], what are the possible available alternatives to quinolones for managing the diseases treated in this chapter, and in particular CBP?
Indeed, the available armamentarium appears to be quite restricted, and evidence from randomized or cohort-observational studies is lacking. For a limited number of drugs, only published case reports or small patient series are available, and many agents must be administered off-label due to the lack of officially approved and registered indications for prostatitis.
For CBP caused by gram-negative pathogens, carbapenems and aminoglycosides, alone or in combination, are possible alternatives to FQs.
The prostate penetration of meropenem has been documented, and sufficient bactericidal concentrations are achieved in the prostate tissue for most pathogens, albeit the antipseudomonal activity of this drug in the prostate is uncertain [44].
For few aminoglycosides (e.g., netilmicin in some European countries), “prostatitis” is an approved indication, and administration is on-label. Adoption of aminoglycosides for long-term treatment of CBP can be hampered by the risk of toxicity to the kidneys and to the inner ear. Whereas renal damage can be sometimes reversible and may be in part avoidable through careful monitoring and continuous patient hydration, cochleotoxicity and/or vestibulotoxicity can appear abruptly and be severe, leading in some cases to profound, irreversible sensorineural hearing loss [46]. The discovery in the late 1990s of the adenine-guanine substitution at the 1555th nucleotide of mitochondrial DNA as the responsible of the onset of hearing loss, often after the very first dose of aminoglycoside [49], was a major scientific breakthrough, and today pharmacogenetic testing for A1555G, C1494T, and other ototoxicity-predisposing mutations may be performed prior to aminoglycoside therapy [6, 63]. In this regard, the Sanford Guide to Antimicrobial Therapy (Table 10A Antibiotic Dosage and Side-Effects) warns clinicians that one in 500 European patients may have mitochondrial mutations that predict cochlear toxicity [22]. Hence, if pharmacogenetic testing is not feasible, careful exclusion of a history of matrilineal deafness is a strongly recommended assessment prior to aminoglycoside administration.
A drug that may be potentially active in the management of a gram-negative prostatic infection is fosfomycin, administered intravenously or orally; evidence of successful resolution of CBP cases was recently presented, though further investigation is warranted [18, 25].
The emergence of enterococcal and staphylococcal strains with multidrug-resistant ability has greatly complicated the management of prostatic infections, and there is an urgent need to assess the efficacy of alternative agents in the frame of adequately designed, powered, and controlled clinical studies. At present (year 2015), the published evidence is scant, and only case reports and case studies are available. For this reason, any recommendation or suggestion is only tentative and based on limited empirical/anecdotal experience.
Gram-positive pathogens like Enterococcus faecalis, Enterococcus faecium, and Staphylococcus aureus (methicillin resistant) are generally sensitive to agents like linezolid and tigecycline.
The oxazolidinone linezolid is officially indicated for treatment of skin, soft tissue, and lung infections, though its activity against fluoroquinolone-resistant uropathogens has been investigated [45, 65]. Linezolid is bacteriostatic against enterococci and staphylococci, but bactericidal for streptococci. It can be administered orally or parenterally, shows a bioavailability of almost 100 %, and the distribution volume equals approximately the total body water (~40 L). The half-life is about 5–7 h, and twice-daily administration is advised [2, 48]. Metabolic acidosis and myelosuppression are among the more severe adverse effects caused by the drug, though in a limited number of cases. Linezolid, administered intravenously (600 mg, twice daily), in combination with oral co-trimoxazole (960 mg b.i.d.), was effective in resolving a complicated case of prostatitis, likely involving both Enterococci and various Enterobacteriaceae [50].
Tigecycline, a glycylcycline structurally resembling tetracycline, can be used to treat gram-positive, but also gram-negative (including metallo-β-Lactamase multidrug-resistant Enterobacteriaceae, but not P. aeruginosa or Proteus spp.) and anaerobic bacterial infections. It is mainly bacteriostatic, but in few cases it is bactericidal, depending on the targeted organism. Its high volume of distribution of 7–10 L/kg suggests diffuse tissue penetration. Moreover, the drug has a conveniently long half-life (about 40 h). A comprehensive analysis of the potential activity of this agent against CBP, together with a detailed case report, has been published by Bates and coworkers [5]. These authors describe administration of intravenous tigecycline, 50 mg twice daily, after a single “kick-in” 100-mg dose, for 6 weeks.
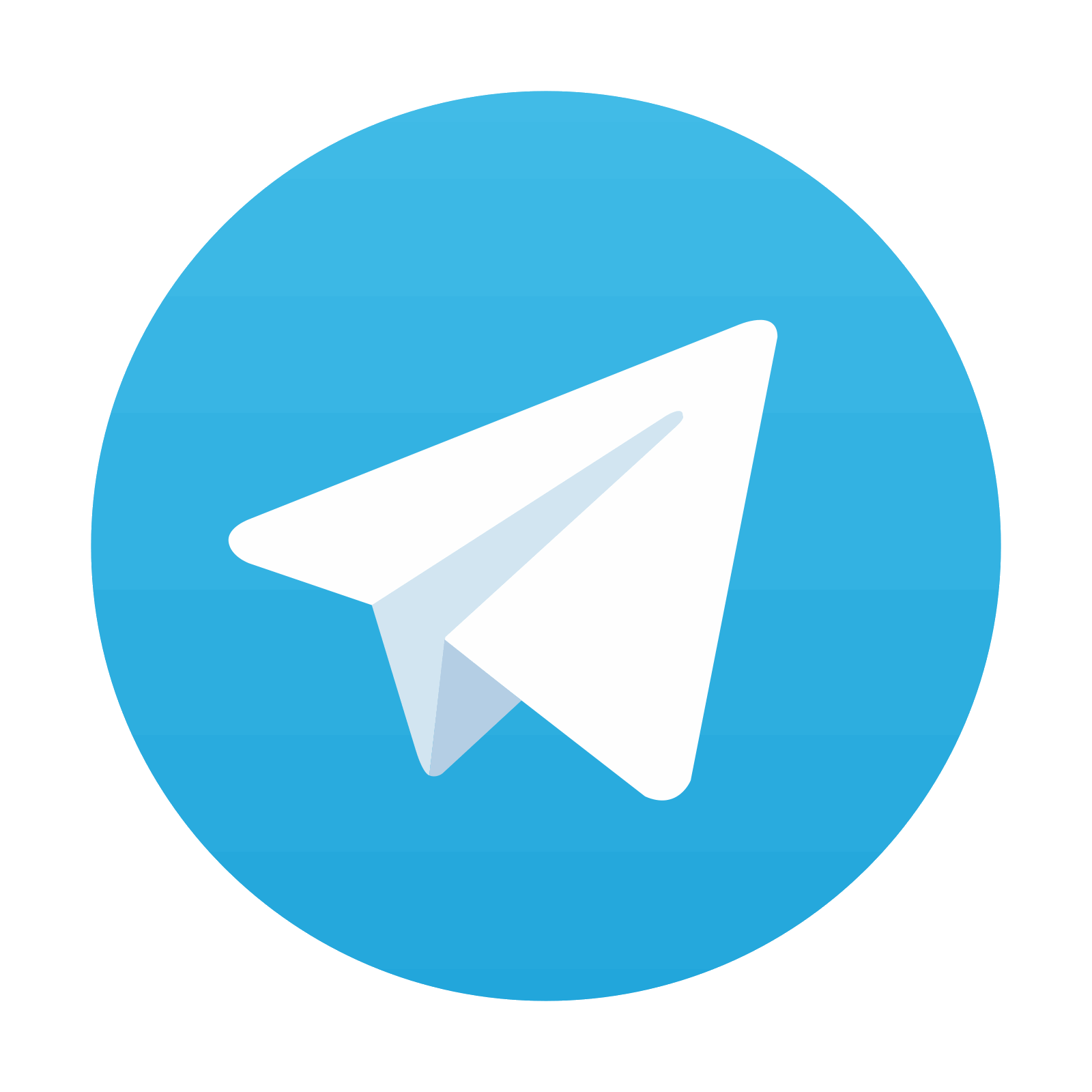
Stay updated, free articles. Join our Telegram channel
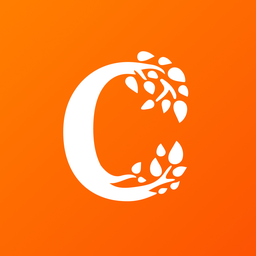
Full access? Get Clinical Tree
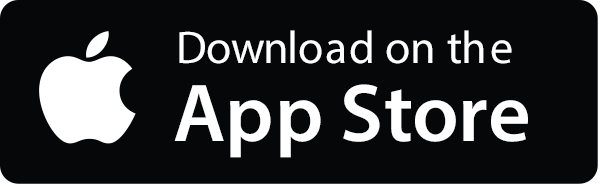
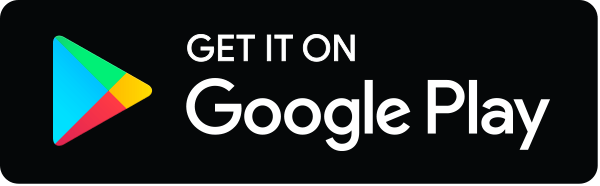