Despite advances in emergency medical systems (EMS) and trauma care, deaths from injury have increased in the United States over the last decade.1 In both the civilian2 and military3 settings, uncontrolled hemorrhage is the leading cause of preventable death after injury. In civilian studies, >95% of deaths from hemorrhage occur within the first 24 hours at a median time <3 hours.2 Consequently, there is intense interest worldwide in the pathogenesis of trauma induced coagulopathy (TIC), and its early management. While there have been substantial insights, the words of Mario Stefanini in his address to the New York Academy of Medicine in 19544 remain applicable today:
“The ponderous literature on the subject of hemostasis could perhaps be considered a classical example of the infinite ability of the human mind for abstract speculation. For several years, the number of working theories of the hemostatic mechanism greatly exceeded and not always respected the confirmed experimental facts. In recent years, however, the revived interest in this field has led to an accumulation of new findings which has been almost too rapid for their orderly incorporation into a logical working pattern. As a result, we have rapidly gone from a state of orderly ignorance to one of confused enlightenment”
Although transfusion medicine has undergone enormous development over the past 60 years since the challenge issued by Stefanini, important gaps in scientific knowledge persist, and several fundamental issues involving the diagnosis and management of TIC remain controversial. The more we learn about TIC the more we appreciate the contributions of surgical scientists from the past century who had the insight to form the basis of our current understanding of trauma-related bleeding. This chapter will refresh important historical landmarks in our evolution of understanding TIC, synthesize recent investigations into the pathophysiology underlying these processes, and provide a rationale for current diagnostic and resuscitation strategies in these patients.
The evolution of our understanding of the complexities of trauma induced coagulopathy has been, in large part, the result of collaboration between civilian and military teams. The early reports of TIC were generated from military research teams, often including civilian consultants, during major wars. These novel observations would then intensify hemostasis research in civilian centers. Ultimately, the resulting findings improved coagulopathy management in subsequent conflicts, and primed the environment for making new observations. The specific contributions to our understanding of TIC, however, are somewhat difficult to ascertain from World War I through Vietnam because the primary focus was on optimizing shock resuscitation at a time when plasma or whole blood was employed to replace acute blood loss.5 Nonetheless, several landmark contributions are well recognized.
In 1916 the US National Research Council formed a subcommittee on traumatic shock that collaborated with the British Medical Research Committee to study wounded soldiers in the front lines of France. Among them was Walton B. Cannon, who was perplexed by the inconsistencies of the prevailing toxin theory of shock. Cannon documented experimentally that stress, that is, epinephrine infusion into animals, provoked hypercoagulability followed by hypocoagulability.6 Cannon stated “ … shock is a loss of homeostasis, and without homeostasis the patient does not survive.”7 During World War II experts recognized that bottled whole blood would be logistically impractical and enlisted the expertise of Edwin J. Cohen, a biochemist, to deconstruct blood to enable components to be used on the battlefield.8 Cohen was successful in purifying albumin as well as preparing plasma. At the onset of World War II, the National Research Council’s Committee on Transfusion recommended that dried plasma—not blood—would be used if combat occurred outside the continental United States because it was easy to prepare and transport; whereas, whole blood had to be typed, cross-matched, and refrigerated. Based on the legendary work of consultant Edward D. Churchill,9 who concluded, “wound shock is blood volume loss,” the policy was changed to whole blood administration and implemented in 1943.
During the 1950s civilian investigators recognized impaired coagulation associated with severe injury; the culprits were believed to be fibrinolysis,10 loss of labile clotting factors,11 disseminated intravascular coagulopathy,12 and platelet dysfunction.13 The labile factor depletion hypothesis was echoed during the Korean War. Scott and Crosby,14 reported that the prothrombin time (PT) was doubled in combat casualties while platelet count and fibrinogen were increased. Artz and Fitts15 observed that severely injured soldiers in the Korean conflict required both return of shed blood and crystalloid for optimal survival. The recognition of the high incidence of acute renal failure further stimulated the classical experimental work of Shires et al16 who demonstrated the accumulation of sodium in hypoxic cells. These studies ultimately lead to the routine practice of initial crystalloid loading for shock resuscitation.17
During the Vietnam war, crystalloid resuscitation was used in such excess that it lead to iatrogenic lung dysfunction, similar to the civilian description of acute respiratory distress syndrome. The factor depletion component of coagulopathy was proposed by Simmons et al18 and attributed to a DIC like phenomena. During the 1970s coagulation research in civilian centers began to accelerate; the recurring theme was trauma patients with liver injury bleeding to death despite control of mechanical hemorrhage. From these challenging patients the concept of pre-emptive plasma resuscitation was introduced19,20; “If the patients remain hypotensive after the second unit of blood—FFP should be administered then and with every fourth unit thereafter” and “fresh whole blood if bleeding persists despite normal PT, PTT, and bleeding times.”20 The bloody viscous cycle, later known as the lethal triad, was introduced in 1981 as it was observed that refractory bleeding was associated with hypothermia, acidosis, and coagulopathy.21 The frequent recognition of this scenario lead to the concept of damage control resuscitation in which definitive repair of injuries was delayed in favor of optimizing the patient’s physiologic status.22 Coagulation research during this period was further complicated by the practice of aggressive crystalloid resuscitation targeting supraphysiologic oxygen delivery. This resulted in an epidemic of compartment syndromes and, in retrospect, much of the coagulopathies were generated by overzealous infusion of crystalloid.23
The first decade of the 21st century represents perhaps the most significant insights into trauma induced coagulopathy in modern history. Progress was unquestionably inspired by the revolutionary concept of the cell-based model of coagulation proposed by Hoffman and Monroe24 who emphasized the fundamental role of platelets as a platform for clotting factor assembly and thrombin generation on damaged endothelium. In 2003, MacLeod et al25 from the University of Miami and Brohi et al from the Royal London Hospital independently reported that 25% of severely injured patients had prolonged clotting times, and this abnormality was independent of fluid administration. Consequently, the London group termed the syndrome, the “acute coagulopathy of trauma” (ACOT).26 Stimulated by his observations of the ACOT, Brohi pursued a trauma research fellowship with Cohen, Pittet, and colleagues in San Francisco. Together, in 2007, this civilian research team provided compelling evidence that activation of protein C was an integral component of ACOT.26 Simultaneous with these provocative civilian findings, the US military analyzed combat casualties in Iraq and concluded that uncontrolled hemorrhage was the predominant source of preventable death.3 Hess et al27 suggested the solution was to replace the lost blood with component therapy that replicated the composition of whole blood, initiating the concept of 1:1:1 (FFP:RBC:platelets). Remarkably in the same year as the protein C hypothesis, Borgman et al28 reported that resuscitation with FFP:RBC approaching 1:1 improved survival. These landmark civilian and military reports prompted a literal explosion of coagulation research. Recognizing the ongoing controversies the Nation Institutes of Health (NIH) responded with a workshop on postinjury hemostasis in 2010, and achieved consensus in renaming this challenge “trauma induced coagulopathy” (TIC).
Hoffman and Monroe24 enhanced our understanding of the mechanisms of coagulation by shifting from a simple enzymatic cascade to a cell-based paradigm with tightly regulated events (Fig. 13-1). The key concept underlying the paradigm of “cell-mediated hemostasis” is that cells play active roles in regulating and localizing the coagulation reactions. Many cells can participate in hemostasis and thrombosis, but the two critical players are platelets and endothelial cells. This model consists of three overlapping phases: initiation, amplification, and propagation. A breach of the endothelium promotes clot formation via exposed collagen and tissue factor. Collagen localizes platelets via their GP-VI receptor. Circulating von Willibrand factor also binds collagen, and this complex further promotes platelet adherence through their GP-Ib-V-IX receptors, particularly important under high-stress flow. Tissue factor (TF) binds circulating factor VII; the resulting TF/FVIIa complex activates factors IX and X. The initial amount of thrombin generated is insufficient to cleave fibrin, but activates platelets through their PAR 1 and 4 receptors and also activates factors V and VIII. In the ensuing amplification phase, these activated factors form the prothrombinase (Va/Xa) and tenase (VIIIa/IXa) complexes on the surface of platelets. Tenase (VIIIa/IXa) generates sufficient amounts of factor Xa to support thrombin generation through prothrombinase (Va/Xa). These coagulation complexes require phospholipid and calcium for their activity. Prothrombinase and tenase then potentiate the “thrombin burst” in the propagation phase, which cleaves fibrinogen. The resulting fibrin polymerizes and integrates platelets by binding via their GP-IIb-IIIa receptors. This thrombin further generates Va and VIIIa for prothrominase and tenase assembly, and activates XIII which cross-links fibrin to stabilize the evolving clot. Activated platelets release adenosine diphosphate (ADP) and thromboxane A2 (TxA2) that further recruit platelets to form an outer shell to stabilize the clot (Fig. 13-2). The various proteins known to be active in the clotting system are summarized in Table 13-1.
FIGURE 13-1
Cell-based model of hemostasis. Initiation is triggered by subendothial TF that binds circulating F VII; the TF VIIα complex activates FX generating thrombin. This initial thrombin activates platelets and FV and F VIII. During amplification the prothrombinase (Vα/Xα) complex generates additional thrombin which activates the tenase (VIIIα/Ixα) complex on the platelet surface. The tenase complex generates sufficient Xα to maintain prothrombinase mediated thrombin which cleanses fibrinogen into fibrin and activates F XIII which cross-links fibrin to form a stable clot. (Reproduced with permission from Gonzalez E, Moore E, Moore H, et al. Trauma-induced coagulopathy: An institution’s 35 year perspective on practice and research. Scand J Surg. 2014;103(2):89-103.)

FIGURE 13-2
Protein C activation. Increased expression of the transmembrane endothelial protein thrombomodulation binds thrombin which activates protein C (A). The endothelial protein C receptor localizes protein, facilitating this activation (B). Activated protein C cleaves peptide bonds of F Vα and F VIIIα preventing their assembly into the prothombinase (Vα/Xα) and tenase (VIIIα/Ixα) complexes.

Protein | Source | Activated by | Function |
---|---|---|---|
Procoagulant | |||
Tissue factor | Subendothelium, monocytes | Binding to circulating platelets | Complexes with VII to initiate clot formation |
Fibrinogen (factor I) | Activated platelets | Thrombin | Clot formation |
Factor V | Activated platelets | Thrombin | Cofactor that accelerates conversion of prothrombin to thrombin |
Factor VII | Liver | Thrombin, Xa, XIa, XIIa | Complexes with TF to convert X to Xa |
Activates TAFI | |||
Factor VIII | Endothelial cell, liver | Thrombin | Cofactor, activates factor X |
Factor IX | Liver | Factor XI | Cofactor, activates factor X |
Factor X | Liver | Factors VIII and IX | Converts ~ prothrombin to thrombin |
Factor XI | Liver | Thrombin | Activates IX |
Factor XIII | Liver | Thrombin | Cross-links fibrin |
Kallikrien | Liver | Activates contact system | |
Anticoagulants | |||
Heparan sulfate | Endothelial cells | Ischemia | Activation of ATIII |
Antithrombin III | Liver | Thrombin | Inhibition of thrombin, Xa, XIa, XIIa |
Protein C | Liver | Thrombin, TM, EPCR | Irreversibly inactivates Va and VIIIa |
Protein S | Liver | Cofactor for protein C | |
Thrombomodulin (TM) | Endothelial cell | Ischemia | Complexes with thrombin to activate protein C; Inhibits TAFI |
Endothelial protein C receptor (EPCR) | Endothelial cell | Complexes with thrombin and TM to activate protein C | |
Tissue factorpathway inhibitor (TFPI) | Liver | Thrombin | Inhibits TF–VII complex from converting X → Xa |
Meizothrombin | Red blood cells | Precursor of thrombin | |
Fibrinolytic system | |||
Plasminogen | Liver | tPA | Converted to plasmin |
Tissue plasminogen activator (tPA) | Endothelial cell | Ischemia, thrombin | Converts plasminogen to plasmin |
Urine-type plasminogen activator (uPA) | Epithelium, monocytes | Kallikrien | Converts plasminogen to plasmin |
Plasminogen activator inhibitor-1(PAI-1) | Endothelium, platelets, adipocytes | Binds tPA and uPA | |
Plasminogen activator inhibitor-2 (PAI-2) | Placenta, adipocytes, monocytes | Binds tPA and uPA | |
Thrombin activatable fibrinolysis inhibitor (TAFI) | Liver, platelets | Thrombin:thrombo-modulin complex | Inhibits plasminogen binding to fibrin |
Α-2 antiplasmin | Liver, platelets | Binds plasmin and inactivates | |
Α-2 macroglobulin | Endothelium, platelets | Binds plasmin and inactivates | |
C1-esterase inhibitor | Liver | Binds tPA and inactivates | |
Platelet activation = adhesion / aggregation / degranulation / shape change | |||
Agonists | Platelet Receptors | ||
Thrombin | PAR-1 and PAR-4 | ||
Collagen | GP1a-IIa and GPVI | ||
Von Willibrand Factor | GP1ba-IX-V | ||
Adenosine Diphosphate | P2Y1 and P2Y12 | ||
Thromboxane A2 | TRα and TRβ | ||
Fibrinogen | GPIIb-IIIa | ||
Epinephrine | |||
Serotonin |
The activated protein C (APC) pathway attenuates coagulation activation, serving as a critical anticoagulant restraint on the multiple physiologic processes that promote clot formation. Independent of these anticoagulant properties, APC has been shown to have multiple cytoprotective effects as well, acting as an anti-inflammatory agent and preventing endothelial barrier leakage.29 By these two mechanisms, the APC pathway serves to maintain vascular flow by preventing excessive thrombosis, and also protects cells from damage associated with inflammatory insults, such as sepsis and trauma. Once activated, APC achieves its primary anticoagulant function through proteolytic cleavage of activated factors Va and VIIIa, the major drivers of thrombin formation.30 Activation of protein C is achieved via thrombin-mediated cleavage.31 Following injury, thrombin binds to upregulated thrombomodulin activating the endothelial receptor for protein C (ERPC) culminating in the release of protein C (Fig. 13-3). Thrombomodulin can increase this activity by 20,000-fold.32 Thrombomodulin is a transmembrane protein that is found predominantly on the surface of endothelial cells, and in heavy concentration in microvasculature.33 Protein S also increases activated protein C complex adherence to the phospholipid membrane, further enabling the more efficient inactivation of factor Va.34 Recently Mann et al have shown that meizothrombin released from stored RBC can also bind to thrombomodulin and activate protein C.35
FIGURE 13-3
Ineffective clot formation is the dominant manifestation of TIC. Hypoxia and tissue injury provoke endothelial dysfunctions which activates protein C and releases anticoagulants from the glycocalyx. Platelet dysfunction, factor consumption, and acidosis further impair thrombin generations and clot formation.

As previously mentioned, data from the San Francisco general group suggested that APC has a central role in pathogenesis of TIC. The hypothesis is that this event is related to an evolved but maladaptive response to severe injury. In the setting of severe trauma and tissue hypoperfusion, an excess of protective anti-inflammatory APC is released in an attempt to prevent local microvascular thrombosis and mitigate cellular dysfunction. This view is supported by data from a trauma cohort demonstrating that poor outcomes associated with increased levels of inflammatory histones are abrogated by simultaneous increases in endogenous APC, implying a protective effect of APC in the setting of widespread inflammation.36
Shortly after the proposed role of APC in TIC, Johansson et al37 from Copenhagen added evidence implicating endothelial glycocalyx degradation, emphasizing the endotheliopathy component of TIC. The vascular endothelium comprises a single layer of cells that line blood vessels and lymphatics in every organ, covering a surface area of 4000–7000 m2 with a weight of 1 kg.38 The luminal surface of the endothelial cells is covered by a 0.2–1.0 μm thick negatively charged carbohydrate-rich surface layer, the endothelial glycocalyx, that represents a large structure in the vascular system by containing a fixed noncirculating plasma volume of approximately 1 L in adults, corresponding to one-third of the vascular plasma volume.38 The glycocalyx is an antiadhesive and anticoagulant structure that protects endothelial cells and maintains vascular barrier function.39 It is bound to the underlying endothelial cells through various backbone molecules of proteoglycans (the most abundant are syndecan-1 and glypican) and glycoprotein. The proteoglycans have long glycosaminoglycan side-chains comprised mainly of heparan sulfate that has heparin-like functions.39 Glycocalyx damage can range from discrete disturbances in the composition of the most luminal layer to degradation with loss of the entire glycocalyx. Upon shedding, the glycocalyx glycosaminoglycans retain their anticoagulant (heparin-like) activity and promote measurable hypocoagulability in the circulating blood through endogenous auto-heparinization.39 Rehm et al40 provided the first evidence of acute destruction of the endothelial glycocalyx in patients undergoing vascular surgery associated with ischemia/reperfusion injury. This study reported that syndecan-1 and heparan sulphate levels increased after ischemia/reperfusion injury during vascular surgery and that this increase correlated to shedding of glycocalyx as detected by electron microscopy. Despite 40- to 60-fold increases in syndecan-1 postischemia, the levels of the classical endothelial adhesion molecules ICAM-1 and VCAM-1 did not change, emphasizing the occurrence of selective glycocalyx disruption. Johansson et al41 translated these observations to trauma when his group demonstrated reversibility of prolonged clotting time by heparinase-TEG in critically injured trauma patients. These patients had syndecan-1 levels four-fold higher than noncoagulopathic patients in this study in addition to a higher injury severity and transfusion requirements.
The role of disseminated intravascular coagulopathy (DIC) in depleting circulating clotting factors resulting in impaired thrombin generation remains debated.42 Theoretically the distinction is that DIC occurs within the vessel lumen; whereas, consumption occurs from disrupted endothelium exposing tissue factor bearing calls and collagen. Most investigators do not believe DIC is a dominant mechanism in TIC, but acknowledge its potential contribution. The innate immune response to injury has been implicated in the genesis of DIC, particularly with the release of damage-associated molecule patterns (DAMPS). But beyond increased tissue factor expression on monocytes and microparticles, the mechanism remains unclear. Among those with procoagulant implications are HMBG1, histone DNA complexes43, and polyphosphates.44 Additionally, neutrophils are believed to contribute to a procoagulant state via degranulation of elastase, which can act as an indiscriminate protease.45 Finally, with extensive tissue disruption, there is undoubtedly some element of coagulation factor consumption to achieve hemostasis.
Hypothermia and acidosis are no longer considered the primary drivers of TIC, but they can become secondary events that complicate the management of coagulopathy. In vitro46 and in vivo47 work suggests that hypothermia affects hemostasis when the temperature is below 33°C. Below this temperature, hypothermia inhibits the initiation phase of clotting. This would be anticipated as most of the coagulation enzymes are slowed by hypothermia. Thus while moderate hypothermia delays the onset of thrombin generation, the total amount of thrombin generation is unaffected.46,47 The effect of hypothermia on platelet function is contradictory due to limitation in the ability to replicated platelet function under physiologic conditions.48 Some studies indicate enhanced platelet activation (priming) and aggregation,49,50 while others indicate decreased adhesion and aggregations.46 Profound hypothermia has been long recognized for promoting hepatic sequestration of platelets, but the clinical relevance outside of cardiac surgery is minimal, as a temperature below 30°C is needed for this phenomenon.
Metabolic acidosis has a more profound effect on TIC than hypothermia at clinically relevant levels.47,51 A reduction of pH from 7.4 to 7.0 has been shown in vitro to reduce FVIIa activity by 90%, prothrombin complex (Xa/Va) activity by 70%, and FVIIa-TF complex activity by 55%.52 In vitro work has confirmed a pronounced inhibition of the propagation phase of thrombin generation at a pH of 7.1, resulting in a marked reduction in clot strength.47 These studies also suggest this degree of acidosis increases fibrinogen degradation two-fold. While impaired platelet aggregation and adhesion at a pH of less than 7 has been appreciated for decades, more recently the mechanism has been elucidated and associated to the store-operated calcium entry (SOCE) channels. Perhaps more worrisome, the correction of pH with bicarbonate47 or tris-hydroxymethylaminomethane (THAM)53 does not restore platelet function. This may have implications for the optimal timing of platelet transfusion in the critically injured patient.
Hemodiution is no longer considered the dominant mechanism for TIC, but overzealous crystalloid resuscitation can exaggerate an existing coagulopathy. Reduced levels of coagulation proteins have been documented in healthy volunteers after administration of crystalloids, colloids, and stored RBCs,54 and remains a fundamental rationale for permissive hypotensive resuscitation (see Chapter 12). Interestingly, acute hemodilution to 50% in vitro does not impair clot formation,55 but this magnitude of hemodilution enhances the sensitivity to tPA due to the dilution of endogenous antifibrinolytics.56
In sum, impaired clot formation is central in the pathogenesis of TIC, but the mechanism is complex and time dependent, and can be exacerbated by hypothermia, acidosis, factor consumption, and hemodilution (Fig. 13-4).
FIGURE 13-4
Systemic fibrinolysis phenotypes. The Y axis represents mortality, the X axis is the fibrinolysis phenotype based on the LY30 measured by TEG. There is a U-shaped distribution of mortality, with a nadir evident in the physiologic group. LY30 above (hyperfibrinolysis) below (fibrinolysis shutdown) this range had increased mortality. (Reproduced with permission from Moore H, Moore E, Gonzalez E, et al. Hyperfibrinolysis, physiologic fibrinolysis, and fibrinolysis shutdown: The spectrum of postinjury fibrinolysis and relevance to antifibrinolytic therapy. J Trauma Acute Care Surg. 2014;77:811-817.)

With the multiple mechanisms that drive TIC, it is not surprising that patients manifest coagulopathy for different reasons, and thus, represent unique phenotypes of coagulopathy warranting patient specific management. Trauma is a combination of tissue injury and shock, and no two patients have an identical injury mechanism and degree of shock. These variables in combination with genetic variability, preexisting medical conditions, and age-related changes in coagulation make every trauma patient unique when they arrive at the emergency department. In the early description of TIC, two components were proposed: (1) impaired blood clot formation (hypocoagulation) and (2) increased clot degradation (hyperfibrinolysis).26 These two processes appear to be mechanistically unique based on principal component analyses, which also implicate a third mechanistic variant of coagulopathy.56,57 To make it more complicated, the extremes of the phenotypes can be pathologic. Recently it has been identified that trauma patients can present with a spectrum of fibrinolysis within 12 hours of injury and those patients on either extreme with excessive or impaired fibrinolysis have increased mortality (Fig. 13-5).58
FIGURE 13-5
Fibrinolysis regulation. Systemic fibrinolysis is controlled conceptually at three levels. The first is direct inhibition of the predominant plasminogen activator tPA. The major inhibitor is the serine protease PAI-1. The next is direct inhibition of plasmin, with antiplasmin the major circulating factor. The third is access to fibrin for degradation via factors that stabilize the clot (F XIIIα) or preventing plasmin binding to fibrin (TAFI). (Reproduced with permission from Moore H, Moore E, Gonzalez E, et al. Postinjury fibrinolysis shutdown: rationale for selective tranexamic acid. J Trauma Acute Care Surg. 2015;78:S65-S69.)

Stafford’s review on fibrinolysis and hemostasis in 196459 established a logical understanding for why coagulation becomes pathologic: “a general assumption has developed that clotting is not episodic but a continuous process which is normally never allowed to progress to a physical end point.” In the previous section we stressed that APC and the gylcocalyx serve physiologic functions to localize thrombin generated clot, and that fibrinolysis serves a physiologic role to control clot burden. The recurring theme of biologic systems becomes apparent in TIC that coagulation is a balance and preventing the extremes should be the resuscitation goal.
Although proposed in 1948,10 during the 1960s a number of investigators emphasized that fibrinolysis was a physiologic process to counter balance thrombosis and was occurring perpetually.60,61,62 Fibrinolysis is the active degradation of polymerized fibrin through the lysine avid binding protease plasmin.63 The conversion of plasminogen to plasmin is accomplished by proteolytic cleavage by one of its activators (tissue plasminogen activator/tPA or urine-type plasminogen activator/uPA). Plasmin activity is highly dependent on its local environment, and its proenzyme plasminogen binds numerous receptors indicating the process occurs on cell surfaces and not in circulation.64 The regional distribution of specialized endothelial cells contributory to the fibrinolytic system is reflective of the importance of localizing this process.65 The primary driver of intravascular fibrinolysis, tPA, is released from precapillary arteriole and postvenular endothelial cells; uPA is considered a secondary source derived from epithelial cells but its relative importance is likely tissue specific. Release of tPA into the circulation results in complex formation with its most abundant serine protease inhibitor, plasminogen activator inhibitor 1 (PAI-1), and secondary inhibitors such as C-1 inhibitor. These tPA complexes are cleared by the liver.66,67 There are a number of additional proteins present in plasma that either inhibit tPA directly, inhibit plasmin, or impair plasmin access to fibrin (Fig. 13-6). These proteins are normally in relatively high abundance in the plasma including α-2 antiplasmin (α-2AP), α-2 macroglobulin, α-1 antitrypsin, and histidine rich glycoprotein.68 In addition there are potent inhibitors integrated into the fibrin clot, including α-2AP and thrombin-activatable fibrinolysis inhibitor (TAFI), which are directly released from platelets to ensure a tight regulation of the system. TAFI cleaves lysine binding sites on fibrinogen, inhibiting plasminogen and tPA binding. Plasma can buffer the effects of tPA in vivo,56 and in vitro exogenous tPA mixed in whole blood of healthy volunteers requires supraphysiologic concentrations to reproduce hyperfibrinolysis.69 While fibrinolysis is constitutively occurring at a microvascular level to maintain vascular patency, pathologic systemic hyperfibrinolysis is the result of not only an increase in circulating tPA, but also a reduction of its circulating inhibitors.
FIGURE 13-6
Clot degradation. Degradation of the clot is an important component of TIC in some patients particularly those with hypoperfusion. Degradation is primarily via the action of plasmin on fibrin, although platelet dysfunction can contribute to clot instability. The mechanisms responsible for postinjury fibrinolysis are complex, and include the depletion of circulating antifibrinolytic agents.

Interest in fibrinolysis as a component of TIC was stimulated by the proposal of Brohi et al26 who suggested activated protein C degraded PAI-1. However, recognition of systemic fibrinolysis following injury did not occur until the widespread use of viscoelastic assays. Recent clinical studies indicate that systemic hyperfibrinolysis occurs in 2–5% of critically injured patient and 10–15% of those requiring a massive transfusion.59,69,70,71,72 In our experience, the highest rate of hyperfibrinolysis in trauma occurs in those undergoing ED resuscitative. Currently, the only established mechanistic factor for hyperfibrinolysis is inadequate tissue perfusion, suggested by a number of retrospective studies59,69,70,71,72 and further strengthened by the fact that the patients in the CRASH II trial who benefited from antifibrinolytics had a systolic blood pressure less than 75 mm Hg.73 Supporting this observation is that blood taken from patients who have nontraumatic cardiac arrest have a high prevalence of hyperfibrinolysis.74 Shock may also generate metabolic disturbances that enhance fibrinolysis. For example, taurocholic acid increases fibrinolysis in vitro and is markedly elevated following shock.75 Recent animal work indicates that tPA levels increase substantially in animals undergoing hemorrhagic shock, while tissue injury does not increase systemic tPA levels.76
The mortality rate of injured patients with impaired fibrinolysis (shutdown) has been reported to be nearly four times greater than patients with a physiologic level of fibrinolysis.73 Death is primarily attributable to organ failure. The term fibrinolysis shutdown was first used in 196977 in a review describing the effects of electroplexy, myocardial infarction, and elective surgery on fibrinolysis. Animal work prior to this time suggested microemboli in visceral small vessels leads to irreversible shock that was later found to be survivable with a profibrinolytic.78 Hardaway further translated these findings to humans and implicated trauma and shock in producing microvascular occlusion.79 Confusion arises as the nomenclature of describing impaired removal of vascular thrombi is often referred to as either the syndrome of disseminated intravascular coagulation (DIC) or pathologic impairment of fibrinolysis (shutdown). As mentioned previously, some investigators consider DIC to have two distinct phenotypes; that is, hyperfibrinolysis and fibrinolysis shutdown,42 which is somewhat difficult to reconcile as diffuse intravascular thrombus should not exist in the presence of systemic hyperfibrinolysis.
It is well known that trauma patients are prone to thrombotic events. The prevalence has been reported to approach 60% when screening for postinjury venous thrombosis.80 There is evidence for thrombosis in the pulmonary vasculature in nearly one in four seriously injured patients within 48 hours of their injury.81 Furthermore, microvascular clot has been implicated in organ dysfunction.82,83 Therefore, it is intuitive that maintaining adequate fibrinolysis to clear the microvasculature of excessive fibrin deposition would be beneficial.
Acute lung injury models using mustard gas have implicated PAI-1 in addition to other antifibrinolytics (α-2AP and TAFI) as culprits in progression to pulmonary failure.82 Fibrinolysis inhibition is more common after trauma than hyperfibrinolysis. In 1991, Enderson et al described that the majority of multisystem trauma patients in their study had elevated d-dimers but low fibrinolysic activity.84 Subsequently, Raza et al85 showed minimal fibrinolysis activity measured by rotational thromboelastometry (ROTEM) and high plasmin-antiplasmin (PAP) complexes in 57% of their patients. The circulating half-life of PAP is 12 hours. Most recently we demonstrated that 65% of severely injured patients had suppressed fibrinolytic activity measured by TEG.60
In sum, enhanced clot degradation (hyperfibrinolysis) (Fig. 13-6) can be a major component of TIC, but the incidence is relatively low and the underlying mechanisms remain unclear. On the other hand, impaired fibrinolysis (shutdown) is present in the majority of severely injured patients and may result in microvascular occlusion and organ dysfunction.
Platelets are anucleated cells fragments from megakaryocyte (MK). Maturation of platelets occurs in two phases.86 The first phase is MK maturation in the bone marrow that takes several days. In the second phase mature MKs grow pedicles that project into the sinusoids of bone marrow blood vessels and fragment into circulation as platelets, a process that only requires several hours. The circulating count of platelets in normal subjects is around 250,000/mcL with a half-life of 9 days, representing a turnover rate of 35,000 platelets per day.
Successful hemostasis requires the rapid accumulation of platelets to occlude the site of blood extravasation (Fig. 13-7). In animal models, platelets can seal a small wound in ~30 seconds, and within minutes form a fibrin rich core.87 Contracted platelets free of fibrin have decreased permeability. This work suggests that activated platelets serve as the primary sealant with fibrin acting as a secondary stabilization of the platelet plug. In addition, platelets form a complex around fibrin clots that provides a unique microenvironment that is both prothrombotic and antifibrinolytic.88,89 Therefore platelets have multiple roles in initiating and maintaining hemostasis. This interplay of platelet auto and paracrine signaling from release of granules and the local environment optimize clot formation and resist degradation.
FIGURE 13-7
Role of platelets in hemostatic thrombus formation. (A) This is a laser-induced injury in a mouse cremasteric arterial, showing platelet and fibrin accumulation. The injury site is indicated and dashed white lines outline the vessel wall. Platelets are labeled with an anti-C41 antibody (red) and platelets that have released their alpha granules are labeled with anti-P selection antibody. Fibrin is labeled with an antifibrin antibody (blue). White represents overlap. (Image used with permission of Timothy J. Stalker, PhD, University of Pennsylvania). (B) The cartoon illustrates platelet incorporation into an assembled hemostatic clot.


Endogenous activators of platelets have been well characterized in both the arterial and venous system with a focus on collagen and thrombin that work in conjunction with platelet released ADP and thromboxane. However, the precise roles of these platelet activation pathways during TIC are not well defined. Interestingly, the TIC patient is more likely to display platelet dysfunction as opposed to platelet consumption.90,91 Platelet dysfunction occurs early after injury, and has been documented with isolated traumatic brain injury.92 In the context of TIC, the molecular definitions of the desensitized, stunned, inactive, postactivated, dysfunctional, degranulated, or exhausted platelets are still emerging. For example, damage associated molecular pattern molecules (DAMPs) have been shown to bind the toll-like four receptor of platelets and promote coagulopathy.93 Circulating platelets may experience combinations or sequential exposures of agonists to trigger modes of activation as indicated by shape change, degranulation, and receptor expression, activation or shedding.
Lack of an accurate tool to identify and track coagulopathy remains a major limitation surrounding both postinjury hemostatic derangements and empiric blood component replacement therapy. Traditional laboratory tests of coagulation function, such as PT and PTT, were designed originally for the assessment of coagulation function with isolated factor deficiencies in hemophiliacs, and are based on the interaction of the coagulation factors in isolation. To date, the performance characteristics of these tests in the trauma patient remain unproven. Furthermore, a prohibitive amount of time (30–45 minutes) is required to conduct these assays. Because both the PT and PTT are performed on platelet-poor plasma, they are sensitive only to the earliest initiation of clot formation (estimated to represent 5% of the thrombin generation during clot formation) and do not incorporate platelet function. Finally, these tests are performed in an artificial environment, irrespective of the patient’s core body temperature and pH. Measurements of individual clotting factors and related proteins, such as protein C, are both costly and time consuming. Diagnosis of fibrinolysis is also problematic. The euglobulin lysis time is a complex and time-consuming procedure that can take more than 180 minutes, reduces some of the inhibitors, and does not include platelets that regulate fibrinolysis. Other techniques suggested to identify hyperfibrinolysis, such as plasmin–antiplasmin complex, fibrin monomers, and d-dimers, are only reflective of the footprint of fibrinolysis; that is, they do not represent the patient’s current systemic fibrinolytic activity. Thus, partitioning the components of a patient’s blood and testing them independently in an artificial environment that requires a lengthy assay time is not clinically optimal to manage coagulopathy in the critically injured patient.
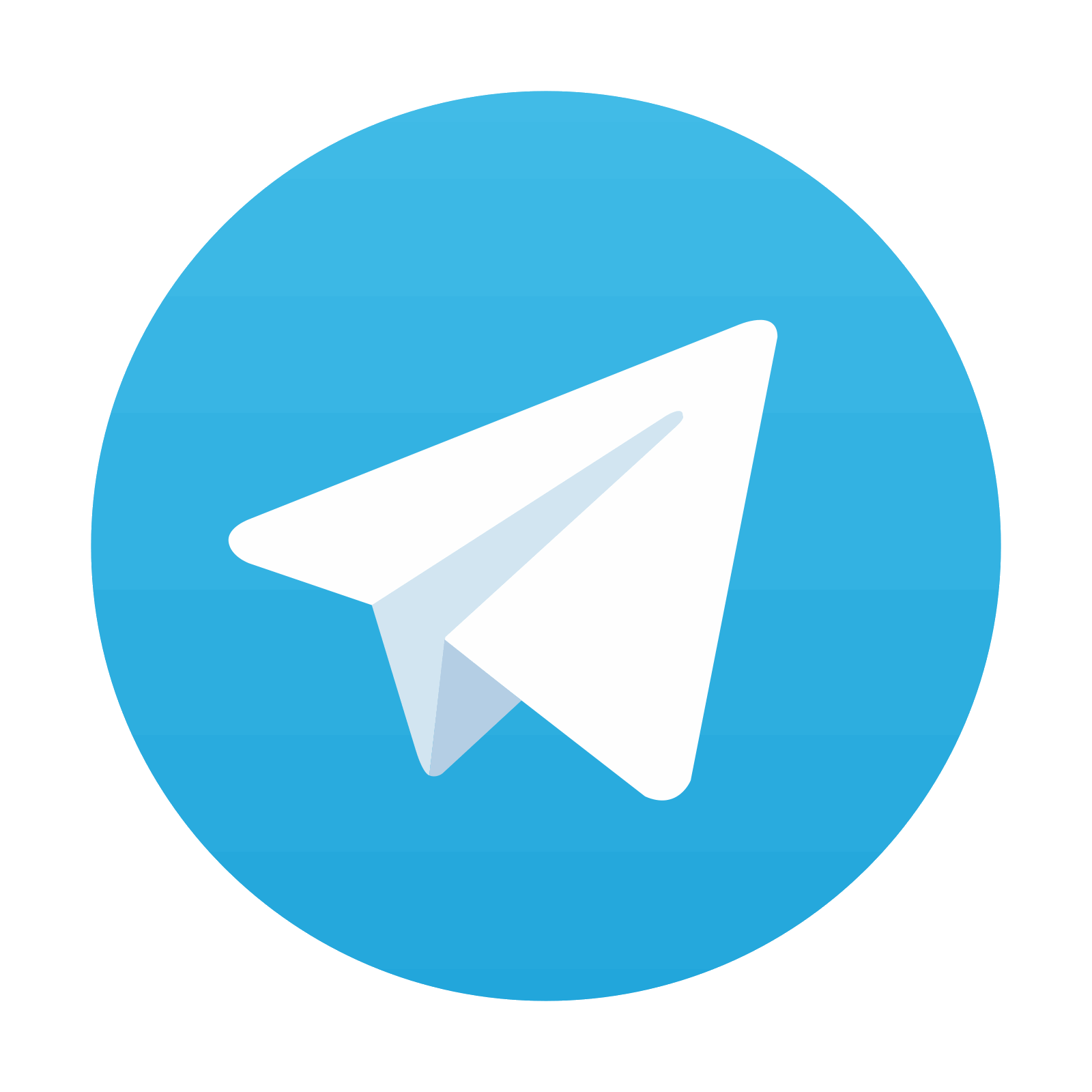
Stay updated, free articles. Join our Telegram channel
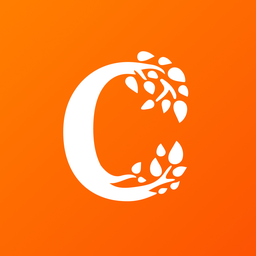
Full access? Get Clinical Tree
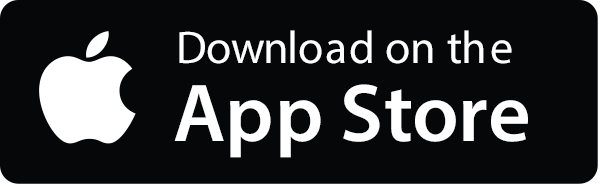
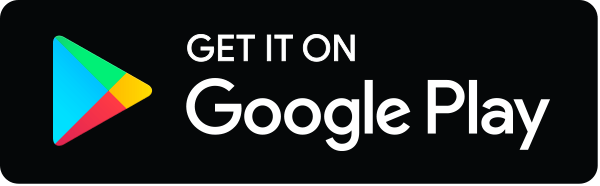