Abstract
The regulation of gastric HCl secretion by the parietal cell occurs primarily through the regulated recycling of H,K-ATPase. Secretagogue stimulation of the parietal cell initiates HCl secretion by effecting an intracellular signaling and biochemical pathway that culminates with the membrane fusion of intracellular H,K-ATPase-rich tubulovesicles with, and thus, the delivery of the H,K-ATPase to the secretory canaliculus (apical membrane). On withdrawal of the secretagogue, cessation of secretion occurs concomitantly with the retrieval of the H,K-ATPase from the secretory canaliculus, and the reformation of the tubulovesicular membrane compartment. The H,K-ATPase resides quiescently in this compartment until another round of secretion is initiated. Intracellular signaling pathways, cytoskeletal elements, and vesicular trafficking machinery must be coordinately regulated to effect this membrane-recycling pathway. This chapter summarizes key findings from experimental approaches taken toward the elucidation of the mechanism of gastric HCl secretion, through the use of electron microscopy, electrophysiology, membrane biochemistry, molecular biology, cell biology, and atomic structure, in the characterization of the regulation of activity of the H,K-ATPase itself, to that of associated ion channels and membrane transporters, as well as of the regulation of H,K-ATPase trafficking.
Keywords
Parietal cell, H,K-ATPase, Tubulovesicle, Microvilli, Membrane recycling, Secretory canaliculus, Ezrin
38.1
Cellular Plasticity of Gastric Acid Secretion
38.1.1
Organization of Epithelial Cells in the Stomach
The stomach is organized by five concentric tissue layers: (1) the mucosal epithelium, lining the lumen of the stomach; (2) a thin layer of smooth muscle called the muscularis mucosae; (3) the submucosa consisting of connective tissue and blood vessels; (4) the tunica muscularis, composed of two layers (inner circular and outer longitudinal) of smooth muscle; and (5) the serosa, facing the peritoneal cavity ( Fig. 38.1 ). The digestive juice is secreted from mucosa in which several types of secretory epithelial cells make up organized tubular gastric glands. The luminal surface of the gastric mucosa is covered by a layer of columnar epithelial cells, the surface mucous cells, which secrete mucus and bicarbonate. It has been proposed that they play a role in protecting the surface from direct acid exposure. The luminal surface is studded with numerous invaginations, or pits, that serve as conduits for secretions from the subadjacent gastric glands.

In mammals, gastric glands are composed of three major types of secretory epithelial cells: mucous neck cells, chief cells, and parietal cells. Mucous neck cells are small cells located in the region of transition at the base of the pits extending throughout the neck of the glands. Mucous neck cells secrete a mucous glycoprotein distinct from that of surface epithelial cells. They also serve as a differentiating precursor to the chief cell as they migrate toward the base of the glands. Chief cells are the principal source of pepsinogen, the inactive precursor form of the protease pepsin, and are abundantly distributed at the base of the glands. The large acid-secreting parietal cells are found throughout the length of the gland, but tend to be more abundant in the neck region of the gland. Because of their large size and relative abundance, parietal cells represent about 50%–60% of the mass of the secretory mucosa. Subadjacent to the glandular epithelial cells, there are a number of endocrine and paracrine cells that play important regulatory roles in gastric secretory function, such as gastrin-secreting G-cells and somatostatin-secreting D-cells. The cellular separation and specialization of acid secretion from pepsinogen release in mammals is an evolutionary landmark as the gastric glands of lower vertebrates (birds, reptiles, amphibians, and fish) use a single epithelial cell, named the oxyntopeptic cell, to secrete both acid and pepsin.
Gastric epithelial cells undergo dynamic renewal to orchestrate tissue homeostasis and digestive physiology. All gastric epithelial cells originate from a small population of pluripotent stem cells located in the upper neck region of the glands that represent the progenitor cells for differentiating into all functionally distinct epithelial cell types in gastric glands. These stem cells are the precursors of the constantly renewing gastric epithelium, which must be sustained by continued regeneration, differentiation, and migration both up the pit to differentiate into surface epithelial cells, and down the length of the gland to replace secretory cells. Each of the gastric epithelial cells has distinct turnover kinetics, or life span. Studies of 3 H-thymidine incorporation into cells of the mouse stomach provide an estimate of average cell turnover times. Because of their direct luminal exposure, surface epithelial cells have a relatively short life of about 3 days. Mucous neck cells spend about 40 days in the neck region of the gland, and as they migrate to the base, they transform into chief cells that have an average life span of about 250 days. Parietal cells have an average turnover time of 71 days. Newly differentiated parietal cells progressively migrate from the neck toward the base of the gland. Morphologic and functional criteria suggest that secretory activity may be downregulated as parietal cells age/migrate. Judging by their apical membrane dynamics and corresponding secretory activity, the younger cells that are in proximity to the luminal surface appear to be more active in HCl secretion than the cells located more basally in the gland.
The existence of multipotent stem cells in gastric glands has been inferred by elegant clonal marking studies, and recent characterization of differentiation markers for the fundic and antral regions of the human and mouse gastric mucosa, as well as the development of ex vivo organoid cultures will facilitate the characterization of the mechanisms underlying gastric epithelial stem cell dynamics and lineage. The leucine-rich repeat-containing, G-protein-coupled receptor 5 (Lgr5) has been established as a gastric epithelial stem cell marker in the gastric antrum. Lgr5 was expressed at the base of prospective corpus and antral glands, whereas expression in the adult was predominantly restricted to the base of mature antral glands. Lineage tracing revealed these Lgr5-positive cells to be self-renewing, multipotent stem cells responsible for the long-term renewal of the antral epithelium. Single Lgr5-positive cells efficiently generated long-lived organoids resembling mature antral glands in ex vivo culture. In addition, Lgr5 together with another stem cell marker, CD44, are expressed at high levels in proliferating mouse gastric organoids that are enriched for a stem cell-like niche for fundic stem cells. When these proliferating organoids are cocultured with immortalized stomach mesenchymal cells, they develop into organoids that contain all of the major cell types of the in vivo gastric gland: surface epithelial, mucous (neck), stem, parietal, chief, ECL, and other endocrine cells. Such organoids can be then used as an in vitro system to study gastric physiological function, such as acid secretion, gastric disease, epithelial cell biology, and tissue repair and regeneration.
In an exciting development for the field of human gastric epithelial cell physiology, human pluripotent stem cells have been differentiated from early-stage three-dimensional gastric posterior foregut spheroids into either fundic or antral mucosal gastric organoids in a Wnt/beta-catenin-dependent fashion. The differentiation of these spheroids into fundic organoids was dependent upon the activation of the Wnt/beta-catenin pathway, and disruption of this signaling pathway resulted in the differentiation of antral organoids. On the basis of the expression of markers of cellular differentiation, both types of organoids contained surface mucous cells, mucous neck cells, and endocrine cells for ghrelin, somatostatin, and serotonin. Gastrin-secreting endocrine cells, as well as the transcription factor Pdx1, were expressed exclusively in antral organoids, while histamine-secreting endocrine cells, parietal cells, and chief cells were expressed exclusively in fundic organoids. The transcription factors Irx2, Irx3, and Irx5 were highly expressed in the differentiating fundic organoids compared to antral organoids. In addition, only the fundic organoids displayed glandular budding morphogenesis. Thus, this system represents a potentially powerful in vitro model for the characterization of the development and physiology of the human fundus, as well as to be able to interrogate human diseases that are not easily recapitulated in animal models, such as H. pylori infection. Finally, human organoids are becoming increasingly useful platforms for drug discovery, development, and screening.
38.1.2
Parietal Cell Morphology and Ultrastructure
The parietal cell has several distinctive morphologic features that are fundamentally significant to function. The cell is generally pyramidal in shape with tight junctions surrounding the apex of the pyramid. In the resting, or non-secreting, cell, there is a limited area of apical membrane in direct apposition to the gland lumen, but more extensive luminal contact is provided by distinctive invaginations of apical plasma membrane deep into the cytoplasm, forming elaborate secretory canaliculi characteristic of parietal cells ( Fig. 38.2 A). The entire apical surface, including the canaliculi, is lined by short, stubby microvilli in the non-secreting state. A cross section shows that the parietal cell cytoplasm abounds with tubular and vesicular membrane profiles, especially prominent in the apical pole, the system of so-called tubulovesicles (TVs). Three-dimensional reconstruction indicates that many of the TVs are, in fact, flattened and more cisternal in shape. The gastric proton pump responsible for gastric acid secretion is the H,K-ATPase, clearly the most abundant protein in this enormous pool of tubulovesicular membranes. Numerous large mitochondria occupying 30%–40% of the cellular mass are also characteristic of the parietal cell, consistent with the energy requirements and high oxidative capacity of these cells. The most remarkable aspect of parietal cell morphology is its ability to undergo massive morphologic rearrangement in response to the stimulation. Although limited by the optics of light microscopy, careful histologic examination allowed Golgi to illustrate the enlargement of canaliculi of secreting parietal cells in the late nineteenth century. Modern electron microscopic analyses have shown that within a few minutes of adding stimulants there are obvious changes in the apical plasma membrane lining the canaliculi. Parietal cell microvilli grow longer and more elaborate with a concomitant disappearance of TVs from the cytoplasm. The process of apical membrane expansion continues until the steady state of secretory activity is achieved ( Fig. 38.2 B). The maximally stimulated parietal cell has an apical membrane surface area that is 5–10 times that of the resting cell. Morphometric studies show that membrane surface area is conserved; the large apical plasma membrane expansion is quantitatively matched by the disappearance and decrease in the surface area of cytoplasmic TVs. Parietal cells retain this “secreting” morphology and sustain HCl secretion as long as the stimulus is applied and metabolic competence is maintained. Fig. 38.3 depicts the general morphologic change in going from rest to maximal secretion, and returning to the resting state. Removal of the secretagogue leads to a dramatic remodeling of the canalicular spaces, followed by a complex process of reincorporation of the apical surface, and therefore H,K-ATPase, back into tubulovesicular forms of the resting cell. The structural reorganization of the recovered cell has been documented; however, little is known about the forces and precise mechanisms that operate this massive recovery. The recent development of super-resolution fluorescence microscopic analyses, such as photoactivation localization microscopy and structured illumination microscopy, or even improvements with image-processing capabilities in confocal microscopy, combined with cell-type-specific genetic manipulation will allow illustration of the molecular dynamics underlying this massive membrane recovery process.


38.1.3
Membrane Dynamics During the Secretory Cycle
It has been proposed that the membrane-recycling hypothesis accounts for the mechanistic basis for the observed morphologic transformations to the functional output of hydrochloric acid in gastric parietal cells. In non-secreting cells, the H,K-ATPase is retained in a quiescent state in cytoplasmic TVs. This has been shown in biochemical analysis of isolated membranes and in intact cells by immunodetection of H,K-ATPase. Stimulation elicits a series of intracellular signaling events that orchestrate the translocation of TVs through a protein machinery involving TV docking, priming, and fusion, ultimately expanding the apical canalicular surface with H,K-ATPase-rich membrane and resulting in the secretion of voluminous, isotonic HCl. This chapter examines the experimental evidence that led to the proposition of this membrane-recycling model and recent advancements that illustrate the molecular details of the membrane-recycling model.
38.2
Molecular Mechanism of Hydrochloric Acid Secretion
38.2.1
H,K-ATPase: The Primary Gastric Proton Pump
38.2.1.1
Identification of H, K-ATPase as the Proton Pump in Gastric Parietal Cells
The biochemical nature of the gastric proton pump was first identified as unique K + -stimulated ATPase and K + -stimulated phosphatase activities in microsomal vesicles that were purified from homogenates of gastric mucosa. The microsomal vesicles are, in fact, parietal cell TVs purified on the basis of size and density. In microsomal vesicles from a non-secreting stomach, K + -stimulated ATPase activity also requires K + ionophores for maximal stimulation, and it was concluded that the isolated TVs have limited K + permeability and an internal K + activating site. Thus, the K + -stimulated ATPase was linked to HCl secretion on the basis of its location in the acid-secreting region of all vertebrate stomachs and its coincident expression with the functional onset of HCl secretion in early development.
38.2.1.2
Functional Transport by the H,K-ATPase
The use of pH electrode and pH-sensitive fluorescent dye techniques linked the K + -stimulated ATPase activity of gastric microsomal vesicles to vectorial H + and K + transport with H + pumped into the vesicles in exchange for K + transport out. The pump was shown to be an electroneutral H + for K + exchange and became known as the H,K-ATPase. The pump-leak model as depicted in Fig. 38.4 A indicates that ATPase-dependent accumulation of H + by the vesicle critically depends on the relative permeabilities of the three principal ions: K + , H + , and Cl − . The interrelations between the pumps and leaks of ions could be observed experimentally and predicted mathematically from Nernst-Planck ion flux predictions and Michaelis-Menten enzyme kinetics. Thus, the H,K-ATPase is the source of gastric H + , operating as a cation exchange ATPase, using the hydrolysis of ATP to transport H + in exchange for K + , with a stoichiometry of 1H + :1 K + :1 ATP under physiological conditions.

38.2.1.3
Membrane Dynamics Associated with HCl Secretion
The pump-leak model carefully describes the interrelations among ATP utilization, proton pumping, and ionic dependencies in the isolated TVs, but there was an important factor missing for operating the pump in a functional parietal cell. The TV experiments required an exogenous K + ionophore, for example, valinomycin. What was the equivalent mechanism in vivo? The answer came from experiments contrasting ATPase and pump activities in vesicles derived from resting and actively secreting stomachs. The first surprise was that H,K-ATPase activity disappeared from the small TV fraction and transferred almost quantitatively to larger, denser membrane vesicles, which are called stimulation-associated (SA) vesicles. More importantly, the SA vesicles pump protons with almost all of the same characteristics as resting TVs, except that they operate independent of a K + ionophore. In fact, the SA vesicles possess relatively selective ion channels: both K + channels and Cl − channels ( Fig. 38.4 B). Comparison of the protein patterns of SA vesicles with TVs demonstrates some differences in composition (see Fig. 38.4 C). Both fractions are enriched in H,K-ATPase, but several additional protein bands are clearly obvious in SA vesicles, including prominent bands for actin and ezrin (apical cytoskeletal proteins discussed below), and several other proteins. These data clearly indicate that SA vesicles are derived from the apical plasma membrane of stimulated parietal cells: they are much larger and more structurally complex than TVs, they contain actin microfilaments and other apical cytoskeletal elements, and they possess K + and Cl − channels consistent with apical membranes. These observations thus provide the basis for pump operation in vivo, as well as the means to link the enzymatic and transport data with the ultrastructural changes associated with secretory activation. A current model of parietal cell activation and HCl secretion is shown in Fig. 38.4 D, placing H,K-ATPase in the context of the membrane-recycling hypothesis. In the resting cell, the cytoplasmic compartment is full of TVs that are rich in H,K-ATPase, but are functionally inactive because of low K + (and Cl − ) permeability. Secretagogue stimulation of the cell leads to recruitment of TVs to the apical membrane providing the source for membrane expansion and placing the pump in a functionally relevant position. K + and Cl − channels in parallel with the proton pump provide the necessary pathways for ionic transfer, with K + recycling through channel and pump as net H + and Cl − are secreted together with complementary osmotic flow of water. Removal of the secretagogue leads to re-sequestration of the membrane and pumps back into the cytoplasmic compartment and with return to the resting state. Exactly how the apical channels are activated and recruited to the apical membrane with stimulation is the subject of much current research, and more detailed discussion about the regulation of these channels is provided later in this chapter.
38.2.1.4
Biochemistry and Structure of H,K-ATPase Kinetics
The gastric H,K-ATPase belongs to the P-type family of transport ATPases, which also includes the ubiquitous plasma membrane Na,K-ATPase and the Ca-ATPase. There are also H,K-ATPase isoforms other than gastric, including those performing H + and K + homeostasis in mammalian renal tubules and colon, and amphibian urinary bladder. In addition to a great deal of structural homology, the family of P-type cation transport ATPases shares the characteristic that their transport cycle depends on phosphorylation, dephosphorylation, and defined phosphoenzyme intermediates, thus designated P-type. P-type ATPases also exhibit two phenomenologically and structurally discrete conformations, designated E 1 and E 2 , which have distinct kinetic variables, such as affinities for substrates. Thus, P-type ATPases are also called E 1 –E 2 ATPases. The overall catalytic and transport cycle for the H,K-ATPase is made up of a series of partial reactions, as shown schematically in Fig. 38.5 . The initial kinase activity is an autophosphorylation step in which the γ-phosphate of ATP is transferred to the aspartate residue D387 (in the P domain discussed below), forming an acyl-phosphate intermediate, E-P. The phosphoenzyme has two conformer states, and their transition involves the release of a proton. One conformation (denoted by E 1 -P in Fig. 38.5 B) has high reactivity with ADP (and low reactivity with K + ), and thus can easily reverse itself; it comprises what is known as the ATP-ADP exchange reaction. Transition from E 1 -P to the second conformation, E 2 -P, is essentially an irreversible step involving the release of a proton to the luminal surface, which is important for the prevention of the reverse reaction of the transport cycle and proton back flow into cytosol. Subsequently, binding of luminal K + to the phosphozyme participates in formation of E 2 -P*K + and promotes another important enzymatic function, the K + -catalyzed dephosphorylation, or phosphatase activity. By this reaction, inorganic phosphate is liberated from the enzyme, which can then resume the phosphorylation cycle once K + is released to the cytoplasmic surface, K + in . The scheme in Fig. 38.5 B indicates that ATP can bind with either the free enzyme (E) or the K + -bound enzyme (E · K). The association of K + is not a simple, rapidly reversible, ionic bond, but rather is described as an occluded form, similar to that described for Na,K-ATPase. It turns out that the affinity of ATP for free E 1 is much higher than for E 2 · K. Thus, one of the rate-limiting steps in H,K-ATPase turnover is the rate of K + release from E 2 · K which, interestingly, is promoted by a high ATP concentration. Because the enzyme is embedded in a membrane with distinct sidedness for specific binding sites, the H,K-ATPase cycle provides vectorial transport of ions. As the concentration of K + in the cell is relatively high, ATP concentration in the millimolar range is necessary for the rapid conversion of E 2 · K to E 1 · ATP and high pump turnover. This is the plausible reason for the high sensitivity of H + secretion to a compromised metabolism or gastric blood flow.

38.2.1.5
Subunit Structure
The H,K-ATPase is made up of two transmembrane subunit peptides designated α- and β-subunits. The available data suggest that the functionally assembled H,K-ATPase is an oligomer of two closely associated (αβ) 2 heterodimers. The 110 kDa α-subunit contains the catalytically active sites such as the E-P phosphorylation site, and 10 membrane-spanning segments, in which amino acids involved in ion binding are located in the M4, M5, M6, and M8 transmembrane segments. The α-subunit has an estimated 73% of its mass residing on the cytoplasmic side of the membrane. The 32 kDa β-subunit has only a single transmembrane segment with more than 80% of its peptide mass, which also includes six or seven sites of N-linked glycosylation, residing on the extracellular side of the membrane. Although the β-subunit does not directly participate in ATP utilization, it is important for stability and “protection” of the holoenzyme. For example, precise folding of the enzyme during synthesis and targeting to the appropriate membrane compartment have been attributed to the β-subunit. Moreover, it has been proposed that the β-subunit may help to provide an answer to the age-old question of why the stomach does not digest itself. A combination of extensive, unique, β-subunit glycosylation and bonding forces within the extracellular domain of the enzyme are proposed to offer protection from the acidic and proteolytic conditions on the luminal aspect of the cell. The short cytoplasmic tail of the β-subunit with ~ 32 amino acid residues contains a tyrosine-based signaling sequence (FRHY) that appears to be important for membrane retrieval and recycling under secretagogue control. The cytoplasmic tail of the β-subunit also interacts with the α-subunit, and seems to prevent the E 2 P conformation as mentioned in Section 38.2.1.6 .
In the highly purified TV fraction, H,K-ATPase is the overwhelmingly predominant protein. The α-subunit is seen as an intensely stained and focused band at about 95 kDa by sodium dodecyl sulfate-polyacrylamide gel electrophoresis (SDS-PAGE), whereas the heavily glycosylated β-subunit runs as a poorly stained broad band extending from 60 to 80 kDa ( Fig. 38.4 C). When oligosaccharides are removed, the deglycosylated β-subunit is nicely focused at its core peptide size of 32 kDa.
38.2.1.6
Molecular Structure
The oligosaccharides on the β-subunit clearly serve important stabilizing functions, but their very presence and especially their heterogeneity have offered a significant barrier until recently to forming high-quality crystals of H,K-ATPase that are suitable for crystallographic analysis. However, Toyoshima and colleagues have succeeded in producing high-resolution structural maps of the closely related Ca-ATPase from sarcoplasmic reticulum, which does not contain a β-subunit, in many different conformations that cover almost the entire reaction cycle. As the enzymes are undergoing the catalytic cycle, there are distinctive structural changes associated with the E 1 and E 2 conformations, and phosphorylation and dephosphorylation. The motional changes occur in the functional cytoplasmic domains associated with nucleotide binding (N domain), phosphorylation (P domain), and “actuation” (A domain), accompanied with the binding and release of transporting ions to (or from) their binding sites in the membrane-spanning segments. There are also E 1 -E 2 motional shifts within the 10 transmembrane segments that serve as a transmembrane channel and predict selective ionic binding sites from the cis – or trans -side of the membrane, depending on the stage in the turnover cycle. Based on the conserved structural and functional similarities, the 3-D structures of both Na,K-ATPase and H,K-ATPase were predicted by homology modeling.
Finally, crystal structures of Na,K-ATPase in a state analogous to E 2 · 2 K + · P i (prepared in the presence of a phosphate analog, MgF 4 2 − ) were presented. Independently, the 3-D structure of H,K-ATPase in a pseudo-E 2 P conformation was also determined by electron crystallography of two-dimensional crystals (prepared in the presence of AlF and ADP). It should be noted that, in this conformation, the N-terminal cytoplasmic region of the β-subunit is in direct contact with the P domain of the α-subunit, stabilizes the E 2 P state, and prevents the reverse reaction from E 2 P to E 1 P. This interaction seems to prevent reverse the flow of protons into the cytosol. Recently, the 3-D structure of H,K-ATPase bound with SCH 28080, which is a K + -competitive H,K-ATPase inhibitor, was also determined by electron crystallography of two-dimensional crystals (prepared in the presence of BeF and SCH 28080), and consequently, the binding site of this drug was identified near the transmembrane segments 4, 5, and 6, in the luminal cavity (see Fig. 38.5 A and Section 38.2.2 in detail). Future crystal structures in more different forms will unveil the successive conformational changes of gastric H,K-ATPase in detail.
38.2.2
H,K-ATPase Chemical Inhibitors
Identification of the gastric H,K-ATPase provided an important target for the development and pharmacologic testing of drugs to regulate and inhibit the acid pump. Generically, the drugs are called proton pump inhibitors (PPIs), and they have been extremely successful in clinical application as well as in studying the mechanics of pump function. A group of compounds described as substituted benzimidazoles was first suggested as antisecretory drugs via the inhibition of H,K-ATPase. In the late 1980s, one of the substituted benzimidazoles known as omeprazole was launched clinically as an acid secretory antagonist. Several additional analogs have since been introduced (e.g., lansoprazole, rabeprazole, and pantoprazole), and are now widely used for treatment of peptic ulcer disease, heartburn, gastroesophageal reflux disease (GERD), and Zollinger-Ellison syndrome. Clinical use of the substituted benzimidazoles has virtually eliminated the need for gastric surgery (except for gastric carcinoma) that was so common before 1990. Fig. 38.6 A shows the structure of a typical substituted benzimidazole PPI in its neutral inactive form and in the acid-activated state. The generic molecule consists of a benzimidazole ring connected to a pyridine ring via a sulfoxide-containing chain; substitutions at the various R positions on the rings contribute to the specific differences among the PPIs. Fig. 38.6 B depicts a series of chemical reactions, ultimately leading to the inhibition of H,K-ATPase. In their neutral form, the PPIs are stable and highly membrane permeable; therefore, the commercial drugs are enterically coated for “protection” from gastric acid so that they reach the intestine for absorption. The reason the PPIs are targeted at the stomach is based on their structure as a weak base. They are protonated by acid and actually “trapped” and efficiently accumulated within highly acidic spaces of secretory canaliculi. Now, exposure to acid within the canaliculus catalyzes activation of the PPIs to a sulfonamide, which is the form of the drug that reacts with cysteine sulfhydryl (SH) groups on the luminal-facing aspect of the H,K-ATPase, thus inactivating the pump. The disulfide bond formation is a covalent modification, but it can be reversed in vitro by strong reducing compounds. The most likely and common site of reaction is Cys813, although certain PPIs (e.g., pantoprazole) may also bind to Cys822. There remains, however, debate about whether the disulfide product can be reduced and reversed in vivo, or whether H,K-ATPase must be replaced by the de novo synthesis of the pumps. The latter may also be a function of the specific PPI and some additional reaction to a non-reducible bond. So, why are the PPIs so specific for the gastric proton pump? In fact, the “specificity” is more a function of simple chemistry and local environment than a high specific binding affinity, which is usually the case for receptor blockers.

Alternative types of H,K-ATPase inhibitors are known functionally as K + -competitive acid blockers (P-CAB). There appear to be several classes of these compounds, exemplified by SCH-28080, AHR-9294, and TAK-438 (vonoprazan fumarate), which can be characterized as hydrophobic cations that compete with K + , and, unlike omeprazole, they are rapidly reversible. These drugs have proven valuable for insight into pump mechanics. SCH-28080 competes directly with K + in binding to the E 2 -P form of the H,K-ATPase, preventing dephosphorylation of the enzyme. Amino acid residues involved in binding with SCH-28080 were identified in the transmembrane segments 4, 5, and 6 by site-directed mutagenesis in combination with homology modeling. A recent crystallographic study identified the SCH-28080 binding site near transmembrane segments 4, 5, and 6 in the luminal cavity and showed that the drug induces a conformational change of H,K-ATPase, resulting in a luminal-open conformation. Unfortunately, many of these drugs were not clinically useful because of unwanted side effects. However, TAK-438 has been used for the treatment of peptic ulcer disease, heartburn, GERD, and Zollinger-Ellison syndrome. It exhibits rapid onset of action and prolonged control of intragastric acidity.
38.2.3
Apical Channel Proteins Directly Supporting HCl Secretion
Physiologists generally accept H,K-ATPase as the principal gastric proton pump, operating as an electroneutral one-for-one H + for K + exchange pump. However, the electroneutral exchange might appear to be inconsistent with earlier studies on intact gastric mucosal preparations suggesting an electrogenic proton pump. Quantitative models of pump operation demand that channels for K + and Cl − be available for efficient HCl secretion ; thus, electroneutral exchange must be coupled to some conductive pathway(s) to account for polarization phenomena noted in intact tissue. In the model of ion transport proteins and pathways within parietal cell membranes, the arrangement of K + and Cl − conductive pathways are shown operating parallel to an electroneutral H,K-ATPase ( Fig. 38.7 ). Although the pump is electrically neutral, the entire system is electrogenic and can be expressed as a mathematical model. The K + channel provides a path for K + to enter the secretory lumen of the parietal cell, where it is recycled by the H + -K + pump back to the cytoplasm. A Cl − channel provides a means for Cl − to enter the secretory canaliculus, representing a counter-ion for K + current across the apical membrane and balancing the charge for net HCl secretion. In the absence of Cl − , the apical membrane behaves as a potential electrogenic H + pump. Thus, the existence of K + and Cl − channels in the stimulated apical membrane, as implied from functional studies of the SA membranes, account for observations on purified gastric vesicles and as electrophysiologic data in situ.

38.2.3.1
K + Transport Across the Apical Membrane
On the basis of location, relative abundance, specific properties, and results of genetic knockout studies, several distinct potassium channels have been implicated as the apical K + conductance associated with acid secretion. KCNQ1 (also known as KvLQT1) was the first K + channel suggested for apical K + recycling in human and rat parietal cells. KCNQ1 is abundantly expressed and apparently colocalizes with H,K-ATPase. Furthermore, acid secretion was inhibited by Chromanol 293B, a presumed specific KCNQ1 channel inhibitor, leading to the proposal that KCNQ1 and its functional subunits, KCNE2 or KCNE3, represent the critical apical K + channel to satisfy the apical K + recycling needs in parietal cells. The stimulation of acid secretion was greatly reduced in KCNQ1 as well as in KCNE2 knockout mice (consistent with a block to K + recycling activity), and, significantly, acid secretion in the KCNQ1 knockout mice was restored when high K + concentrations were applied to the luminal solution (where there is no need to recycle K + for acid). Thus, KCNQ1 is a reasonable candidate for the apical K + channel. Further studies suggested that KCNQ1 may be localized to subpopulations of TVs distinct from those that contain H,K-ATPase and that KCNQ1 may be trafficked independently from the H,K-ATPase to the canalicular membrane upon stimulation of the parietal cell, although data from another study suggested that most of the KCNQ1 remained in a cytoplasmic pool upon stimulation, as shown in biochemical and immunofluorescence microscopic experiments.
A recent study using knockout mice of the KCNJ1 (Kir1.1 or ROMK) K + channel suggested that KCNJ1 may also play a crucial role in gastric acid secretion and functions together with the KCNQ1/KCNE2 channel to recycle K + across the apical canalicular membrane of the parietal cells.
Fujita et al., used RT-PCR and immunostaining to demonstrate that KCNJ10 (Kir4.1), a member of the inward rectifying K + channel family, is also expressed in parietal cells with specific localization to apical microvillar regions. In a separate study, KCNJ10 was shown biochemically and by immunofluorescence microscopy to translocate to the canalicular membrane upon secretagogue stimulation, and KCNJ10 and the H,K-ATPase were shown to co-immunoprecipitate from both resting and stimulated cells. Alternatively, Malinowska et al., proposed yet another K + channel candidate to facilitate gastric secretion. They found an abundance of mRNA for KCNJ2 (Kir2.1), and immunofluorescence microscopy confirmed KCNJ2 expression in parietal cells, apparently colocalizing with H,K-ATPase and ClC-2 Cl − channels. Western blots showed that KCNJ2 was present in H,K-ATPase-rich membrane vesicles isolated from stomach in either the resting or stimulated state. Channels from vesicles incorporated into planar lipid bilayers did not show rectification, but rather had linear current-voltage relationships with a relatively low slope conductance. The open probability for membranes derived from the stimulated stomach was greatly increased compared with the resting stomach. Treatment of resting vesicles with PKA and/or reduction of pH tended to increase open probability of incorporated channels toward that of stimulated vesicles, thus prompting the suggestion that the channel is regulated in a manner parallel to gastric ClC-2 Cl − channels.
Western blot analysis showed that a fourth K + channel, the KCNJ15 (Kir4.2) K + channel, is highly expressed in the stomach, and a biochemical study suggested that KCNJ15 is translocated from intracellular vesicles to the apical membrane upon stimulation and may be responsible for apical K + recycling. Interestingly, KCNJ15 is apparently localized in vesicles distinct from the H,K-ATPase-enriched TVs in resting parietal cells.
At this time, it is not possible to commit to any one of the apical K + channel candidates. It is known that certain tissues express a diversity of K + channels. In fact, it may be naïve to expect that a single K + channel could account for the many requisite properties to accomplish HCl secretion. The channel must operate at enormous variations of pH and membrane voltage; furthermore, there are questions of activation, such as by membrane recruitment or by some direct channel activation, such as phosphorylation, to account for variations in secretory output. In addition, it is not clear whether and how significantly necessary is quantitative co-translocation and co-localization of the H,K-ATPase and a K + channel in a stimulated cell. Given the high turnover rate of channel conductances, it is possible that a limited number of any K + channel(s) could adequately support maximal rates of H,K-ATPase activity. Clearly, however, there will likely be one or more K + channels that operate to maintain the apical recycling pool of K + for H,K-ATPase activity.
38.2.3.2
Cl − Transport Across the Apical Membrane
In parietal cells, Cl − efflux across the apical membrane is necessary for HCl secretion. So far, as with the case of K + channels, several Cl − channels and transporters have been suggested as candidates that could be involved in the luminal Cl − efflux.
A chloride channel type 2, called ClC-2 protein, has been identified in apical membrane vesicles prepared from stimulated rabbit parietal cells. These ClC-2 channels are regulated by protein kinase A (PKA) (in rabbits and humans, but not in mice). Recently, Nighot et al., showed that ablation of ClC-2 in mice results in reduced stimulated acid secretion in accordance with reduced parietal cell number, reduced TVs, and reduced H,K-ATPase. However, there has been some controversy regarding the expression of ClC-2 protein in rabbits, and no significant expression of ClC-2 protein was observed in the gastric mucosa of rats and humans. In addition, in contrast to the study mentioned above, ClC-2-deletion mice were also reported to show no abnormalities in gastric acid secretion. Although the differing phenotypes of the ClC-2 knockout mice with respect to gastric HCl secretion might be potentially due to the genetic backgrounds of the mice, the exact role of ClC-2 in gastric acid secretion remains uncertain.
SLC26A9 is a member of the solute carrier 26 transporter family (SLC26) and functions as a Cl − channel and Cl − /HCO 3 – exchanger. Xu et al. reported that SLC26A9 deletion results in decreased gastric acid secretion and the loss of TVs at a young age and reduction in the number of parietal cells in adult mice. They suggested that it plays an essential role in HCl secretion by regulating Cl − secretion and/or by affecting the biogenesis of TVs and/or secretory canaliculi in parietal cells.
The cystic fibrosis transmembrane conductance regulator (CFTR) is expressed in TVs prepared from the hog stomach. Secretagogue-induced H,K-ATPase activity in isolated gastric glands from mice could be significantly reduced by either glibenclamide or another chemical inhibitor of CFTR, CFTR-inh172. In addition, mice expressing CFTR harboring the most prevalent mutation that causes cystic fibrosis in humans (ΔF508) were found to have significantly blunted acid secretion in response to secretagogue in both isolated gastric glands as well as whole stomach preparations. On the other hand, another study reported no defect in HCl secretion in CFTR knockout mice.
Parchorin (CLIC6) has significant homology to the family of chloride intracellular channels (CLIC), some members of which, including parchorin, appear to reside in cytosolic as well as membrane-associated pools. One member of the family, CLIC1, has been shown to possess anion channel activity in planar bilayer lipid membranes. CLIC6 is distributed throughout the cytosol of the parietal cells. Treatment of parietal cells with secretagogues results in translocation of CLIC6 from the cytosol to the plasma membrane in association with Cl − efflux ; thus, its involvement in the regulation of Cl − transport has been only indirectly demonstrated. However, given the role of CLIC4 in regulating apical exocytosis and luminogenesis in renal epithelial cells, this family of channels remains an intriguing candidate for regulating Cl − secretion, and also perhaps exocytosis of the H,K-ATPase, in parietal cells.
The chloride-proton exchanger ClCN-5 is also expressed in the TVs of gastric parietal cells. ClCN-5 was co-immunoprecipitated with H,K-ATPase, and its function as a Cl − channel appeared to be regulated by its interaction with the H,K-ATPase.
Electroneutral K + -Cl − cotransporters (KCCs) belong to a cation-chloride cotransporter gene family (SLC12). KCC4 is one of the KCCs, and it is predominantly expressed, and associated with the H,K-ATPase, at the apical membrane, but not in TVs of gastric parietal cells. KCC4 can increase H,K-ATPase activity by effectively supplying K + to the luminal surface of the H,K-ATPase as well as function as a Cl − -transporting molecule for basal HCl secretion in resting parietal cells, although effects of KCC4 deletion on acid secretion have not been examined yet.
At present, similar to the situation with K + channels, it is not possible to commit to any one of the apical Cl − transport candidates. The mechanism of Cl − secretion across the apical canalicular membrane may be more complex than anticipated, with possibly multiple Cl − channels and transporters mediating HCl secretion.
38.2.4
Basolateral Transport Proteins That Contribute to Parietal Cell Secretion
In addition to apical membrane transporters, the parietal cell is endowed with basolateral membrane transport proteins that support acid secretion and maintain cellular homeostasis. As there is a net H + secretion from serosa to mucosa, so must there be an equivalent and opposite flow of base (HCO 3 − ). Moreover, pathways for Cl − entry must be available to balance net trans-tissue Cl − flow as HCl, as well as what constitutes active Cl − transport even in the absence of acid secretion. Finally, the basolateral membranes of all cells contain regulatory systems to balance critical ionic conditions, for example, cytoplasmic pH and Ca 2 + .
38.2.4.1
Na + /H + and Cl − /HCO 3 – Exchange Pathways
Studies with isolated gastric glands and probes to measure intracellular pH (pH i ) have demonstrated that a large portion of NaCl uptake is based on independent, electroneutral Na + /H + and Cl − /HCO 3 – exchangers. When parietal cells were depleted of K + and loaded with Na + , and then resuspended into Na + -free media, pH i decreased. Conversely, when cells were first acid loaded, the rate of pH i recovery was promoted by the presence of Na + in the extracellular medium, but not by K + or impermeant cations. These changes in pH i , in response to changes in Na + concentration, were blocked, or slowed down, by amiloride, an inhibitor of Na + /H + antiporter, or exchanger (NHE). After blockade by amiloride, the changes in pH i could be mimicked by the exogenous addition of a Na + /H + exchange ionophore. Similar experimental evidence supports the existence of Cl − /HCO 3 – exchange activity. For example, replacement of serosal Cl − by impermeant anions induced an increase in pH i ; this increase was rapidly reversed on the reintroduction of Cl − . The Cl − -dependent changes in pH i were abolished by treatment with anion exchange inhibitors (stilbene disulfonates, DIDS), and then were reestablished by the introduction of “artificial” Cl/OH – exchange by adding tributyltin. The Cl − /HCO 3 – anion exchange isoform known as AE2, a member of the SLC4 family, is expressed at high levels on the basolateral membrane of parietal cells, and knockout of the AE2 gene in mice results in achlorhydria. Parietal cells of the AE2-null mice also exhibited a severe deficiency in the development of secretory canaliculi, even though the AE2 expression normally occurs at the basolateral membrane. Certain functional properties of basolateral Cl − /HCO 3 – exchange in parietal cells differ from the known functional properties of AE2, raising the possibility that other basolateral Cl − /HCO 3 – exchanger(s) may be present to play a role in bicarbonate exit. A Cl − /HCO 3 – exchanger belonging to the SLC26A transporter family has been identified in gastric tissue. One family member, known as SLC26A7, is prominently expressed on the basolateral membrane of parietal cells. Animal experiments demonstrated that deletion of the chloride transporter SLC26A7 causes distal renal tubular acidosis and impairs gastric acid secretion. It will be of interest to ascertain the extent to which SLC26A7 participates in support of acid secretion and the exchange of intracellular HCO 3 – for extracellular Cl − .
Basolateral Na + -H + exchange is important for pH homeostasis and to assist in driving Cl − into the cell. Three Na + -H + exchanger isoforms (NHE1, NHE2, and NHE4) are expressed in parietal cells; therefore, they could contribute to couple Na + -H + and Cl − /HCO 3 – exchange at the basolateral membrane as well as pH i regulation. However, NHE1 is expressed at relatively low levels in parietal cells, and analyses of NHE1 knockout mice indicated that this isoform is not essential for acid secretion. Mice having a knockout of the NHE2 gene exhibited a progressive loss of parietal cells and became achlorhydric as they aged; however, gastric secretions in young NHE2 knockouts had normal acidity. Thus, NHE2 seems to be essential for parietal cell survival, and studies in isolated parietal cells demonstrated the involvement of NHE2 in pH i regulation, but NHE2 does not appear to be directly involved in acid secretion. The NHE4 isoform is most abundant in parietal cells and functionally appears to be more sensitive to hyperosmolarity than to pH i ; thus, it has been proposed to be more important in the regulation of parietal cell volume than pH i and, accordingly, driving Cl − into the cell. The occurrence of a hypochlorhydria phenotype in the NHE4 knockout also supports this hypothesis. Thus, the Na + -H + and Cl − /HCO 3 – exchange activities, involving NHE4 and AE2, with secondary coupling to the Na,K-ATPase, are major pathways for electrolyte uptake and maintenance of cell volume and membrane potential during gastric acid secretion.
The ubiquitous Na,K-ATPase (Na pump) is an important transporter at the basolateral membrane of parietal cells, principally because of the fundamental properties of the Na pump to maintain the electrochemical gradient of cations, such as low cellular [Na + ] and high cellular [K + ], in opposition to high [Na + ] and low [K + ] in the serosal fluid. Inhibition of the sodium pump by ouabain or by removal of serosal K + leads to the diminution of gastric acid secretion. These observations are explained by the resulting depletion of cellular K + and consequent inhibition of K + recycling and the H,K-ATPase at the apical membrane. Addition of high K + to the mucosal bathing solutions restores acid secretion, because this supplies the H + pump with the necessary K + for exchange.
The basolateral membrane is also endowed with a carbachol-induced Ca 2 + permeability pathway. In isolated canine parietal cells exposed to cholinergic agonists, free cytosolic Ca 2 + was observed to increase when Ca 2 + was present in the extracellular medium and decrease when Ca 2 + was absent. From their studies with isolated parietal cells, gastric glands, and isolated membrane vesicles, Muallem and Sachs concluded that a calmodulin-dependent Ca-ATPase was also present in the basolateral membrane. This Ca pump, and not a Na-Ca exchanger, was proposed to be responsible for the efflux of Ca 2 + in the steady state. In view of some pharmacologic similarities and current information from a variety of cellular systems, it is tempting to speculate that the increased K + conductance observed with cell stimulation reflects the existence of Ca-dependent K + channel in the basolateral membrane.
38.2.5
Na-K-2Cl Cotransport
The Na + -K + -2Cl − (NKCC1) cotransporter is expressed in several gastric mucosal cells, including parietal cells. NKCC1 cotransporters move Na + , K + , and Cl − , with water following osmotically, into the cell. The NKCC1 isoform is present in the basolateral membrane of the parietal cell, where, coupled to the Na pump, it is thought to function in the maintenance of intracellular Cl − and K + concentrations and cell volume. It has been proposed that NKCC1 participates in acid secretion from studies showing that HCl secretion is dependent on serosal Na + and Cl − and is blocked by Na-K-2Cl cotransport inhibitors such as furosemide and bumetanide. However, for mice in which the gene responsible for NKCC1 expression was knocked out (NKCC1 −/−), secretion of gastric acid was normal, with generally no apparent histologic abnormalities in parietal cells. Interestingly, McDaniel and Lytle reported abundant immunohistochemical staining of NKCC1 in rat stomachs; however, it was predominantly distributed to parietal cells located in the lower half of the gastric glands. Parietal cells in the upper half of the rat glands displayed prominent AE2 staining. These data suggest there may be multiple ways to satisfy the K + and Cl − requirements for parietal cell function and HCl secretion. The AE2 exchanger may be more important in supporting the proton transport activity of the nascent, more active, secretory cells in the upper portion of the gland, whereas the NKCC1 cotransporter may be more functionally involved in active chloride transport and non-acidic secretion in the lower regions of the gland.
38.2.6
Membrane Trafficking Machinery
The dramatic morphological change in parietal cells associated with HCl secretion is mainly due to the fusion of intracellular TVs with the apical membrane. It is generally believed that both homotypic fusion among TVs and heterotypic fusion between TVs and apical membrane occur during parietal cell activation. This transformation is a highly orchestrated process achieved by the collaboration of regulated vesicle trafficking, membrane mixing, and rearrangement of the actin cytoskeleton. Specifically, an array of proteins must coordinate their activities to regulate vectorial trafficking of the H,K-ATPase to the apical membrane and subsequent priming and membrane fusion events, in addition to endocytic processes for H,K-ATPase retrieval after the withdrawal of the stimulus. Despite the highly specialized morphology and function of parietal cells, recent characterization of the cellular machinery responsible for acid secretion in parietal cells has clearly shown that the core components of this secretory machinery are conserved in many other cell types. The following sections describe the identification and characterization of these proteins in parietal cells.
38.2.7
SNAREs in Tubulovesicle Docking and Fusion
The specificity and driving force for membrane fusion have been a fascinating subject in the cell biology of exocytosis, with a great deal of cell biological and biochemical data supporting what has become known as the SNARE hypothesis. SNAREs ( s oluble N -ethylmaleimide-sensitive factor a ttachment protein re ceptors) are a group of membrane proteins that reside on the donor and target membranes of a pair of fusing membranes, at various subcellular locations. SDS-resistant SNARE complexes often form between one SNARE protein on the vesicular membrane (v-SNARE) and several SNARE proteins on the target membrane (t-SNARE). In general, the SNARE protein complex provides a platform for two pools of membranes to interact and integrate.
The initial search for SNARE proteins in the parietal cell identified six SNAREs: VAMP, syntaxins 1, 2, 3, and 4, and SNAP-25. Subcellular fractionation experiments showed that VAMP2 and syntaxin 3, but not syntaxin 1 or SNAP-25, are localized to intracellular TVs. Live cell imaging with GFP-VAMP2 demonstrated the translocation of VAMP2 from TV membranes onto the apical canalicular membrane upon stimulation. The functional importance of VAMP2 in stimulated acid secretion was demonstrated by the treatment of parietal cells with tetanus toxin, a Zn-dependent proteinase, which specifically cleaves VAMP2 and thereby inhibited acid secretion.
While identification of VAMP2 as a v-SNARE in parietal cells may have been expected, the identification of syntaxin 3 on TVs was a surprise. This prototypical plasma membrane t-SNARE is instead localized to vesicular membranes of parietal cells and may mediate homotypic fusion among TVs, accounting for the rapid apical morphological changes associated with active acid secretion. Upon stimulation of parietal cells, syntaxin 3, translocated from TV along with H,K-ATPase to the canalicular membrane. The functional importance of syntaxin 3 in supporting acid secretion was demonstrated with an SLO-permeabilized gastric gland model in which recombinant syntaxin 3 was added to compete for endogenous protein, resulting in a dose-dependent inhibition of 14 C-aminopyrine accumulation. In a phenotype-based screen for critical regulators underlying parietal cell secretion using GFP-syntaxin 3 translocation as a reporter, the cell-cycle dependent kinase cdk5 and Munc18b (Munc 18-2), the mammalian homolog of Sec-1 that is enriched in epithelial cells, were identified as essential components in cAMP-dependent parietal cell activation. Munc18b bears a novel syntaxin 3-selective binding site located at its most C-terminal 53 amino acids. Significantly, the phosphorylation of Thr572 by cdk5 attenuates Munc18b-syntaxin 3 interaction and promotes the formation of a Munc18b/syntaxin 3/SNAP-25 tripartite complex, leading to an assembly of a functional Munc18b/syntaxin 3/SNAP-25/VAMP2 membrane fusion complex. The finding that cdk5-mediated phosphorylation of Munc18b functions in promoting SNARE complex formation together with cdk5’s requirement in secretagogue-regulated gastric acid secretion suggest a novel regulatory mechanism in which Munc18b regulates vesicle docking and fusion in secretagogue-stimulated gastric acid secretion, although the interaction of Munc18 with SNARE complexes is conserved in other systems.
SNAP-25 has been localized to the apical membrane by imaging and subcellular fractionation experiments. Expression of mutated SNAP-25, in which the syntaxin-interacting C terminus was deleted, inhibited acid secretion in parietal cells. In addition, fluorescently labeled SNARE proteins and subcellular fractionation analyses found SNAP-25 and syntaxin 1 on the apical membrane of parietal cells. These results are consistent with SNARE complexes participating in the fusion of TVs to the apical membrane. In an in vitro reconstitution assay, isolated TVs have been shown to fuse readily with each other and even fuse with pure liposomes in response to Ca 2 + or Mg 2 + /ATP added at physiological concentrations. Interestingly, fusion is possible even when one of the membranes does not contain any SNARE proteins and is enhanced when there is a negative membrane surface charge. Taking these results, together with the prevailing evidence supporting a role for SNAREs in parietal cells, we hypothesize that SNARE proteins may have a primary role in the recognition and docking events, but that an additional mechanism, possibly involving insertion of a hydrophobic protein domain from one membrane into a closely apposed membrane, may provide the energy to form a fusion event.
38.2.8
Rab and Arf GTPases
Rab GTPases belong to the Ras GTPase superfamily, which regulates many steps of membrane trafficking. Rabs are found tethered to membranes through two prenyl groups at their C termini, and switch between GDP-bound and GTP-bound forms depending on a variety of GTPase activation proteins (GAP), GTPase dissociation or displacement inhibitors (GDI), and GTPase exchange factors (GEF). Interaction with these proteins leads to the production of GTP-bound Rabs, which then recruit Rab effector proteins for downstream activities. In time, GAPs will activate the Rab GTPase, leading to the hydrolysis of GTP, and a new cycle of Rab activity. Cycling between GTP/GDP-bound and -unbound forms through the action of GDIs and GEFs may provide a temporal and spatial regulation to intracellular trafficking events.
Rab11 is involved in regulating recycling endosomes in transferrin recycling models, apical membrane exocytosis, and apical endosomal recycling in epithelial cells, and is also required for trans-Golgi network (TGN) to plasma membrane trafficking. Initial screening for Rab proteins in parietal cells had identified Rab11 as the most abundant Rab at the mRNA level and localized Rab11 to the H,K-ATPase-enriched TVs. Functional studies in parietal cells expressing the dominant-negative mutated Rab11 N124I resulted in a 60% inhibition in stimulated acid secretion. This inhibition was directly correlated with impaired membrane translocation of H,K-ATPase from TVs to the apical plasma membrane. Identifying Rab11 effectors in parietal cells is essential to unveil the molecular details of regulating membrane-recycling events. The first Rab11 effector, Rab11BP/Rabphilin-11, was isolated and cloned from brain tissue and was later characterized as a TV-associated protein in gastric parietal cells, together with two other Rab11 effectors: Rab11 family interacting protein 1 (Rab11-FIP1), also called Rab-coupling protein (RCP), and Rab11-FIP2. The interaction of Rab11-FIP2 with myosin Vb has shown that Rab11 has a regulatory function for endosomal motility in apically recycling protein cargo in epithelial cells in particular.
Interestingly, both Rab11-FIP3 and FIP4 can form ternary complexes with Rab11 and the ADP-ribosylation factor (Arf) GTPase Arf6. Arf GTPases are conserved regulators of membrane trafficking and dynamics of the actin-based cytoskeleton at the leading edge of migrating cells. Arfs are so named because they were first identified to be protein factors required for cholera toxin to ADP-ribosylate the alpha subunit of the heterotrimeric G protein G s . However, endogenous roles for Arfs proteins do not involve ADP ribosylation or regulation of G s . Like other small ras-like GTPases, Arfs switch between GDP- and GTP-bound states. Again, GEFs are responsible to load ARFs with GTP, whereas GAPs activate GTPase activity, causing the hydrolysis of GTP to GDP. These factors together dictate the timing of Arf6 switching between two states, therefore serving as timing switches for regulating cellular activities. Arf6 is expressed in parietal cells more abundantly than in other gastric epithelial cell types, consistent with the high demand for membrane turnover at the apical membrane. Like the H,K-ATPase, native Arf6 redistributes from a predominantly cytosolic localization to the apical canalicular membrane when cells are stimulated. Heterologous expression in parietal cells of the mutated, Q67L Arf6, which lacks GTPase activity but is functionally constitutively active resulted in the inhibition of acid secretion. Interestingly, addition of GTPγS to permeabilized parietal cells resulted in translocation of Arf6 from the cytosol to TV, but it also inhibited secretagogue-dependent translocation of the H,K-ATPase to the canalicular membrane, suggesting that completion of the GTPase cycle of Arf6 is critical to its effector function and that it is either the predominant small GTPase that regulates H,K-ATPase translocation and HCl secretion or it operates downstream of the main rab GTPases, as GTPγS is typically used to activate rab proteins and their effector function.
A key determinant of Arf6 function is the lifetime of the GTP-bound active state, which is orchestrated by GAPs and GEFs. However, very little is known about the molecular mechanisms underlying Arf6-mediated membrane dynamics in parietal cells. A systematic proteome-wide search for Arf6 regulators had identified a novel Arf6-specific GAP, ACAP4. ACAP4 contains two coiled coils, one pleckstrin homology (PH) domain, one GAP motif, and two ankyrin repeats. Biochemical characterization demonstrated that ACAP4 has a phosphatidylinositol 4,5-bisphosphate-dependent GAP activity specific for Arf6. The co-localization of ACAP4 with Arf6 occurred in dynamic membranes formed upon the addition of aluminum fluoride, which activates small GTPases, or epidermal growth factor (EGF). Ezrin (described later in this chapter) interacts with ACAP4 in a PKA-mediated, phosphorylation-dependent manner in gastric parietal cells through the N-terminal 400 amino acids of ACAP4. ACAP4 interacts with ezrin in a phospho-Ser66-ezrin-dependent manner in vitro and in vivo. ACAP4 localizes primarily to TVs in resting parietal cells but translocates to the apical membrane upon histamine stimulation. Immunofluorescence of fixed cells and real-time imaging analyses suggest that phospho-ezrin specifies the membrane domain for active recruitment of H,K-ATPase-containing TVs and activation of acid secretion. Taken together, these results define a novel molecular mechanism linking an Arf6-ACAP4-phospho-ezrin interaction to parietal cell secretion.
Besides Rab11, other Rab proteins (Rab1a, Rab10, Rab14, and Rab25) are also expressed in parietal cells, but their functional significance has remained elusive. The most recent members of the rab family shown to regulate parietal cell secretion are Rab27a and Rab27b. They were abundantly expressed in stomach parietal and mucus cells in a transgenic mouse expressing a reporter gene fused to the Rab27a and Rab27b initiation codons. Eight Rab27b peptides on highly purified TV membranes were identified by a proteomics approach. Rab27b enrichment on immunoisolated TV membranes was confirmed on immunoblots and by immunofluorescence. Rab27b was shown to be principally associated with H,K-ATPase-rich TV along with Rab11. Native and overexpressed Rab27b translocated to the apical membrane along with H,K-ATPase following stimulation. Overexpression of wild-type Rab27b did not affect acid secretion; however, expression of dominant-negative Rab27b N133I almost completely inhibited acid secretion. The relative roles and importance of all of these rab and Arf GTPases in coordinating HCl secretion by parietal cells remains to be characterized, but the presence of multiple GTPases suggests a complex interaction of trafficking pathways in the regulated recycling of the H,K-ATPase. It is likely that other GTPases will be involved in the regulation of the trafficking of the H,K-ATPase.
38.2.9
Vesicle Coat Proteins and Apical Membrane Remodeling and Recycling: Clathrin, Clathrin-Associated Proteins, and Endolysosomal-Associated Proteins
Although inhibitory signals for acid secretion do exist (e.g., somatostatin and EGF/TGF-α pathways), withdrawal of the stimulus, that is, withdrawal of the secretagogue, alone seems to be sufficient to turn off parietal cell secretion. Withdrawal of the secretagogue leads to a cessation of acid secretion, concomitant with a progressive, massive internalization of the stimulation-associated expanded apical canalicular membrane and re-sequestration of H,K-ATPase intracellularly, as the cytoplasmic tubulovesicular compartment is reformed. Thus, the H,K-ATPase represents the major cargo of internalized canalicular membrane and of the reformation of the tubulovesicular compartment. Indeed, the absence of either the α- or β-subunit of the H,K-ATPase in knockout mice results in the absence of TVs. By their relative abundance in parietal cells, coat proteins, such as clathrin, dynamin, and other clathrin-associated proteins, such as clathrin adaptors, are implicated in these critical processes, and these proteins are discussed below, but the process of internalization of the canalicular membrane, H,K-ATPase, and reformation of the tubulovesicular compartment is incompletely understood.
The formation of a coat at the site of vesicle budding enables the membrane to deform into a pit. The clathrin coat is best characterized among three known vesicle coats (the others are COPI and COPII). The major component of the clathrin coat is clathrin. Each clathrin subunit consists of three heavy and three light chains that together form a tripolar structure called a triskelion, which could spontaneously form polyhedral cages in vitro in the absence of membrane vesicles, suggesting an innate capacity of clathrin to form these cages without a membrane template. Another major component of the clathrin coat is the adaptor proteins, which are also multisubunit protein complexes. Adaptors are the connection between clathrin and membrane proteins, including membrane cargo proteins to be incorporated into the nascent vesicle. Therefore, adaptors are part of the mechanism for cargo selection. There are five types of adaptor proteins for the clathrin coat, AP-1 through AP-5, each interacting with distinct sets of membrane proteins that are cargo to be incorporated into clathrin-coated vesicles. In mammalian cells, clathrin-coated vesicle formation from the plasma membrane is mediated by AP-2, whereas clathrin-coated vesicle formation from the TGN is mediated by the other isoforms of adaptors.
The abundance of clathrin in parietal cells suggests an important role for this protein complex in these cells. Clathrin has been localized to canalicular membranes and TVs, while AP-1 is also localized on TVs as the first identified non-Golgi-associated distribution of AP-1. H,K-ATPase is the major tubulovesicular membrane protein in parietal cells and has been localized by immunoelectron microscopy to coated vesicles ; thus, H,K-ATPase may be selectively enriched in clathrin-coated membranes and vesicles and may interact with clathrin adaptors. The cytoplasmic tail of the β-subunit of the H,K-ATPase contains a tyrosine-based sequence that is predicted to function as a tyrosine-based consensus internalization motif. The physiological importance was demonstrated in a transgenic mouse model in which expression of a mutated β-subunit of the H,K-ATPase, where the tyrosine residue was substituted with alanine, resulted in the apparent constitutive expression of the H,K-ATPase at the canalicular membrane and sustained secretion of acid, leading to the development of gastric ulcers and hypertrophic gastropathy. This construct has been used to understand the overall contribution of trafficking of the H,K-ATPase in HCl secretion and the coordination of trafficking of the H,K-ATPase from accompanying K + channels.
CD63, a tetraspannin membrane protein that also contains a tyrosine-based motif for interaction with clathrin adaptor complexes, co-localizes with the H,K-ATPase in parietal cells. It interacts noncovalently with the H,K-ATPase β-subunit, as shown by their co-immunoprecipitation, and their heterologous co-expression in fibroblast cells changes the subcellular localization of the H,K-ATPase β-subunit from a predominantly plasma membrane distribution to an intracellular, endosomal one, suggesting that CD63 may play a role in regulating the endocytosis and post-endocytotic trafficking of the H,K-ATPase via its interaction with the β-subunit, although it is not clear how their interaction is regulated.
Dynamin is a large, multidomain GTPase, and members of this family of proteins are involved in the regulation of many cellular processes underlying membrane dynamics, including scission of transport vesicles, division of organelles, actin dynamics, and cytokinesis. The PH domain in the middle of the dynamin sequence potentially allows it to interact with phosphatidylinositol 4,5-bisphosphate (PIP 2 ). The C-terminal proline-rich domain interacts with a myriad of SH3-domain-containing proteins that are involved in endocytosis and other cellular activities. Thus, dynamin may regulate membrane dynamics by serving as a scaffolding protein.
Dynamin’s role in endocytosis comes from its unique property of self-assembling into spiral collars around the neck of a budding membrane vesicle in a GTP-dependent fashion, to effect the fission of membrane vesicles. In addition, dynamin independently appears to regulate dynamic actin structures, such as actin comet tails, lamellipodia, filopodia, circular dorsal ruffles, and podosomes/invadosomes. In parietal cells, dynamin was localized to the apical canalicular membrane in both resting and stimulated cells. Like Arf6, dynamin is much more abundant in parietal cells than in other cell types in gastric glands, which again is consistent with needing to regulate large volumes of endocytosis from the apical canalicular membrane. However, the exact role for dynamin in parietal-cell endocytosis or other cellular activities, such as regulating the actin cytoskeleton, has remained uncharacterized and will depend upon the use either of mutated dynamin that constitutively activates or inactivates its GTPase activity or of small-molecule compounds that block dynamin activity, such as dynasore.
Since the last update, new, intriguing proteins involved in trafficking pathways that were previously uncharacterized in the regulated recycling of the H,K-ATPase have been identified and characterized in parietal cells. EGF substrate 15 ( Eps 15) in teracting proteins (epsins 1, 2, and 3) are a family of ubiquitin-binding endocytic clathrin adaptors. Epsins are multidomain-containing proteins comprised of an e psin N – t erminal h omology (ENTH) domain, that directly interacts with the membrane lipid bilayer and deforms it, followed by binding motifs for ubiquitin, the AP-2 clathrin adaptor, clathrin, and E ps15 h omology (EH) domains. They are concentrated at clathrin-coated pits and effect membrane curvature concomitant with the recruitment of ubiquitinated cargo and clathrin. While epsin 1 and 2 have broad organ distribution with high levels of expression in the brain and both are expressed in the stomach, epsin 3 is selectively expressed in the stomach. Epsin 3 localizes to puncta in canalicular membranes of parietal cells where it co-localizes with a subset of clathrin puncta. Epsin 1 co-localizes with epsin 3 in clathrin-coated vesicles in parietal cells. Interestingly, the knockout of epsin 3 is without apparent effect on parietal cell function, suggesting that epsin 1 and 2 may have overlapping functions with epsin 3 in parietal cells. However, analysis of these knockout mice revealed that e psin- h omology d omain (EHD) proteins EHD1 and EHD2 are expressed in parietal cells. EHD proteins are ATPases with membrane tubulating activity and play a role in regulating the recycling of cargo from endosomal compartments to the plasma membrane. Expression of EHD1 and EHD2 in stomachs is elevated in epsin 3 knockout mice, and epsin 1 and epsin 3 interact with EHD1 and EHD2 in biochemical pull-down assays, suggesting a functional interaction between the epsins and EHD proteins. EHD proteins, either with or without epsin, may thus play a role in the biogenesis of the tubular morphology of TVs.
The ubiquitination of proteins was first characterized as a posttranslational modification to target proteins for intracellular degradation via the proteasome. However, it is clear that ubiquitination regulates other intracellular functions, including protein trafficking. TRIM50 belongs to a family of multidomain-containing proteins that possess E3 ubiquitin ligase activity, although for TRIM50, its putative ubiquitin ligase activity has not yet been confirmed. Among major organs such as the brain, lung, heart, stomach, liver, spleen, kidney, muscle, and testis, TRIM50 is selectively expressed in the stomach, and specifically in parietal cells, where it is localized to tubulovesicular and canalicular membranes. In TRIM50 knockout mice, HCl secretion is impaired, although the expression and activity of the H,K-ATPase do not appear to be affected. In addition, TRIM50 appears to be required for the normal progression of the actin cytoskeleton and membrane transformations in the parietal cell from the stimulated state back to the resting state, as the absence of TRIM50 in knockout mice results in a disorganization of the recovery phase with unusual morphology: swollen microvilli lacking F-actin filaments and irregular canalicular membrane and multilamellar membrane profiles. However, it is not clear whether these defects are due to an absence of the ubiquitin ligase activity of TRIM50 or loss of another, ubiquitin ligase-independent, activity of TRIM50. It is interesting to speculate whether there may be a functional interaction with TRIM50, via its ubiquitin ligase activity, and epsin 3 through its ability to bind to ubiquitinated endocytic cargo.
The mucolipins (Trpml or MCOLN) are integral membrane proteins belonging to the Transient Receptor Protein family of cation channels. There are three isoforms of the mucolipins (ML1, ML2, and ML3), all of which localize to endolysosomal membrane organelles, where they are proposed to regulate some aspect of lysosomal function, such as membrane trafficking or protein degradation. The absence of ML1 is the cause of an autosomal recessive neurodegenerative lysosomal storage disease, known as mucolipidosis type IV (MLIV). The symptoms of MLIV are severe mental and psychomotor retardation, diminished muscle tone, visual problems, and, interestingly, achlorhydria and hypergastrinemia. In fact, hypergastrinemia is the hallmark symptom that distinguishes MLIV from other lysosomal storage diseases. Ultrastructural analysis of neurons of the central nervous system either from MLIV patients or from MCOLN1 knockout mice reveals that these neurons possess a relative abundance of large lysosomes that contain multilamellar microgranular inclusions, distinct from the structure of lysosomal contents observed with other lysosomal storage diseases. Interestingly, these types of lysosomes were also observed in parietal cells, both in abundance and structure ; the dysfunctional lysosomal system in parietal cells thus somehow was thought to contribute to the achlorhydria. However, Sahoo et al. have shown that ML1 is a tubulovesicular Ca 2 + channel that mediates a histamine- and PKA-dependent release of Ca 2 + from TVs, and this rise in intracellular Ca 2 + is required for the fusion of TVs with the canalicular membrane. Thus, this work confirms the structural and functional roles of intracellular Ca 2 + stores and fluxes in the regulation of acid secretion by the parietal cell. And, the production of aberrant lysosomes in parietal cells of MCOLN1 knockout mice may be another distinct, yet uncharacterized, consequence of the absence of ML1, although a role for aberrant lysosomal structure and function in effecting achlorhydria cannot be ruled out.
38.2.10
Vesicle Coat Proteins and Recycling of H,K-ATPase: Is it Clathrin-Dependent Endocytosis?
There is indeed evidence supporting the interaction of H,K-ATPase with clathrin and its associated proteins. The disruption of the putative clathrin-dependent internalization motif in the β-subunit of the H,K-ATPase results in its constitutive expression at the canalicular membrane, and the H,K-ATPase is co-localized by immunogold electron microscopy with clathrin-coated vesicles. In addition, there is a biochemical abundance of clathrin in parietal cells. Thus, it would be reasonable to conclude that the re-uptake of H,K-ATPase from the canalicular membrane upon the withdrawal of secretagogue would be a clathrin-dependent endocytotic process. However, clathrin has yet to be observed to be associated with the complicated pentalaminar membrane (presumably endocytotic) structures abundantly observed in the early phases of re-uptake of H,K-ATPase, even under conditions in which clathrin-coated membranes are clearly visible. In addition, the clathrin-dependent retrieval of individual H,K-ATPase molecules seems highly inefficient. Thus, alternative endocytotic pathways may be involved with the massive re-uptake of H,K-ATPase, in conjunction with clathrin-dependent trafficking, such as macropinocytosis or megapinocytosis. Macropinocytosis is an attractive choice because the parietal cell expresses proteins that are involved in phagocytosis/macropinocytosis in other systems, such as dynamin, AP-1 clathrin adaptor, phosphatidylinositol 3-kinase (described later in the chapter), Arf6 (described earlier), myosin IIB (described later), and coronin (described later), and the sensitivity of the re-uptake step to actin microfilament disrupters. In addition, morphological features are shared between megapinocytosis and the re-uptake phase in parietal cells. Thus, the flow of membrane during the re-uptake phase of the parietal cell’s secretory cycle would be first a massive internalization of H,K-ATPase and membrane by a macropinocytotic-like process, followed by a clathrin-dependent, high-fidelity endocytotic and recycling phase. The endocytotic phase would internalize proteins to be localized to TVs that remained at the canalicular membrane after the macropinocytotic step (such as the H,K-ATPase or v-SNARE proteins) and the recycling step would return proteins to the canalicular membrane that may have been inadvertently internalized during the macropinocytotic step (such as K + channels or t-SNARE proteins). Such clathrin-dependent activity occurring during the resting phase is consistent with the abundance of tubulovesicular and canalicular membrane-associated clathrin observed biochemically, by immunofluorescence, and by electron microscopy.
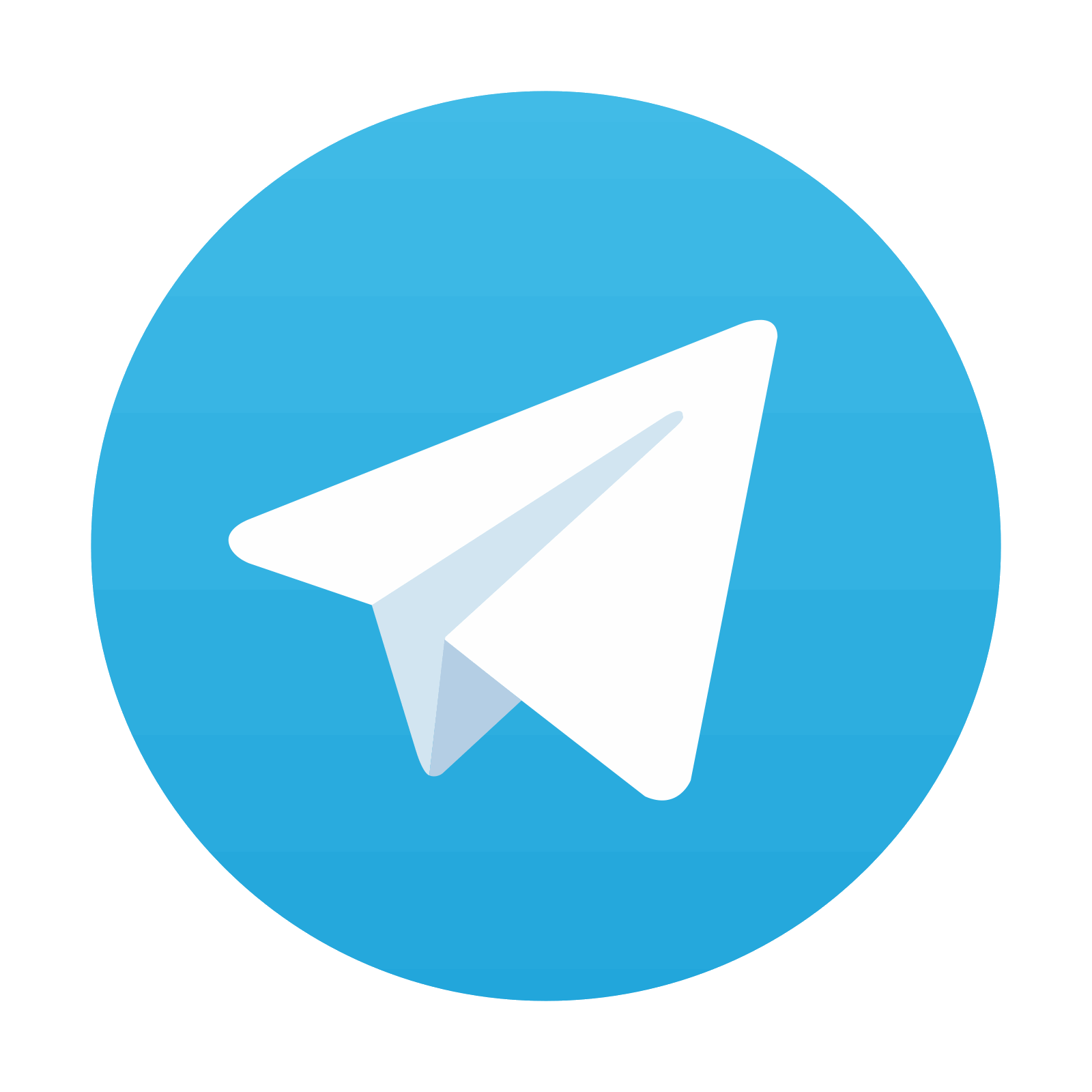
Stay updated, free articles. Join our Telegram channel
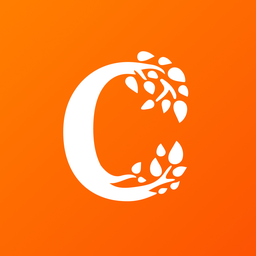
Full access? Get Clinical Tree
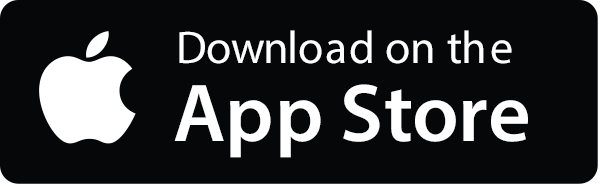
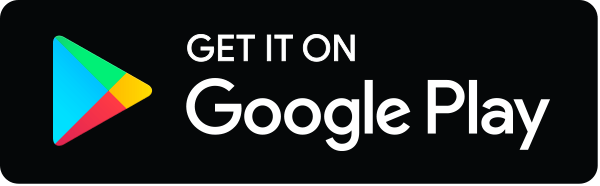