Abstract
Carbohydrates in the diet are digested in the small intestine to glucose, galactose, and fructose, and these sugars are then absorbed by enterocytes lining the upper third of the intestinal villi. Absorption occurs in two steps: first across the brush border membrane, and the second across the basolateral membrane. SGLT1 and GLUT5 are responsible for glucose, galactose, and fructose transport across the brush border membrane, and GLUT2 is thought to be responsible for glucose, galactose, and fructose across the basolateral membrane. Surprisingly, the molecular mechanism of sugar transport through these proteins is quite similar. The physiological importance of SGLT1 and GLUT2 in determining glucose absorption in vivo has been studied using OGTTs in Sglt1- and Glut2 -null and wild-type mice. The results demonstrate that SGLT1 is important for fast glucose absorption, but the presence of GLUT2 is not so critical. SGLT1 plays an important role in water absorption. Given the success in the use of renal SGLT2 inhibitors in treating T2DM, focus has turned to developing SGLT1 inhibitors to control blood glucose levels in diabetes, and some success has been achieved in animal models.
Keywords
SGLT1, GLUT2, GLUT5, OGTT, Water, Diabetes
Acknowledgments
We are indebted to our colleagues and collaborators who have made major contributions to our research on SGLTs, and grants from the National Institutes of Health that provided financial support.
As in animals, humans need to eat, digest, and absorb food to grow and conduct their daily activities. Glucose contained in the diet and synthesized in the liver and other organs is a central unit of energy currency in the body. The concentration of glucose in plasma is held within narrow limits, 4–10 mM, to ensure a steady supply to the organs of the body where it is primarily used to generate the ATP required to fuel the electrical activity in the brain, muscle contraction, secretion, and absorption in the gastrointestinal (GI) tract and kidney. Surprisingly to many, glucose is not an essential nutrient, as the body can meet its needs from gluconeogenesis. Normally, adult humans consume 100–800 g of carbohydrates each day in the form of starches, di- and monosaccharides, subject to availability and individual preferences. Digestion in the GI tract results in the breakdown of complex carbohydrates to glucose, galactose, and fructose before assimilation can begin. In the 6th edition of this work we focus on the recent advances in glucose, galactose, and fructose absorption across the wall of the small intestine, and readers are referred to other chapters in this and earlier editions for discussions about digestion, sugar absorption and metabolism, and the control of food consumption.
46.1
Overview
Fig. 46.1 gives an overview of the steps in glucose absorption and distribution after a test meal. The transit time for food to pass from the stomach to the anus is highly variable, but on average the stomach empties in 4–6 h, digestion and absorption in the small intestine takes 6–8 h, and unabsorbed food remains in the colon 1–3 days. Following delivery to the stomach, the sugar empties into the duodenum (1), where it is absorbed into the blood (2). Before entering the general circulation, glucose passes through the liver, where it may be stored and released (3, 4). In this simple scheme, the liver is included with the other organs (brain, muscle), and the rate of release is determined by metabolism, including synthesis and breakdown of glycogen and gluconeogenesis. Finally, glucose may be lost via the urine through the kidneys (5).

Initially, the test used to study glucose absorption in humans involved drinking a solution containing 2 g of glucose per kg and monitoring blood glucose levels over time, the so-called oral glucose tolerance test (OGTT, see Fig. 46.2 ). However, it has become increasingly clear that it is necessary to consider all the above variables to interpret changes in blood glucose levels in an OGTT. The Cobelli group has led in the use of multiple tracer techniques and modeling to describe the rate of appearance of glucose in plasma after a test meal (R a ), after considering endogenous glucose production (EGP), and glucose disposal (R d ). Their approach has been to include [ 13 C]-glucose as a tracer in the test meal, and estimate EGP and R d from intravenous infusions of [6,6- 2 H 2 ]-glucose and/or [6- 3 H]-glucose in dual and/or triple tracer protocols (see, e.g., Refs. ). For a critical evaluation of the dual and triple OGTTs, see Ref. . The bottom line is that it is not trivial to extract the rate of glucose absorption in vivo in human or in experimental animals.

The approach that we have embraced for OGTTs is to use positron emission tomography (PET) with nonmetabolized glucose tracers. The advantages are that one can monitor continuously the distribution of the PET tracer throughout the living subject as a function of time and determine the rates of gastric emptying, glucose absorption, and distribution throughout the body. The obvious disadvantages are that glucose is ingested as a simple sugar, not a test meal, and that metabolism in the intestine, liver, and other organs cannot be evaluated.
The results of typical OGTTs in mice are shown in Fig. 46.2 . Following an overnight fast, the blood glucose levels were measured before and after gavage of a test meal of 2 g of glucose per kg of mouse body weight. The blood glucose level increased from 3.5 to 12 mM in 40 min and then slowly returned toward the fasting level. When phlorizin, the specific inhibitor of SGLTs, was included in the gavage solution, the rise in blood glucose was blunted. This is taken as evidence for the importance of SGLT1 in glucose absorption in fully conscious mice. Although the primary use of OGTT in the clinic is to evaluate insulin release in response to an oral glucose load, it has limited usefulness in evaluating glucose absorption in the absence of additional information about EGP and R d from double and triple tracers (see above).
After the digestion of complex carbohydrates by pancreatic enzymes to free monosaccharides, chiefly glucose, galactose, and fructose, the sugars are absorbed into the blood by the mature enterocytes on the upper third of the intestinal villi ( Fig. 46.3 ). It is commonly accepted that the monosaccharides are absorbed by transport proteins embedded in the brush border and basolateral membranes, SGLT1 (1), GLUT2 (7), and GLUT5 (6), and that passive absorption through the plasma membranes and tight junctions (2, 4, and 8) is of minor importance. Glucose (and galactose) is actively transported into the epithelium by the sodium-glucose cotransporter (SGLT1) in the brush border membrane (1), and the sodium gradient across the brush border membrane is maintained by the basolateral Na/K-pump. The sugar selectivity of SGLT1 is quite restrictive in that only hexoses with an equatorial ―OH group at carbon #2 are accepted. Large substitutions of ―OH groups are only accepted at C#1 in the beta-conformation (β-glucopyranosides), and these may be either transported substrates, such as indican, or potent competitive inhibitors, such as phlorizin. Fructose is not a substrate for SGLT1 and the sugar is transported across the brush border membrane by the facilitated fructose transporter GLUT5 (6). Glucose and fructose within the epithelium escape into the blood through the facilitated glucose transporter GLUT2 (3 and 7). The net result of this polarized distribution of sugar transporters and the Na/K-pump is the net absorption of glucose, sodium, and water across the intestine and is the basis of oral glucose therapy (OGT) for secretory diarrhea (see below). It has been proposed that GLUT2 may be present in the brush border membrane and play a role in passive glucose and fructose transport. The hypothesis is controversial but we do not think that it is an important player given that glucose OGTTs are normal in mice and humans lacking GLUT2 ( Glut2 -null mice ; Fanconi-Bickel syndrome ).

46.2
SGLT1
Most of the evidence indicates that SGLT1 ( SLC5A1 ) is solely responsible for the active transport of glucose across the intestinal brush border membrane. The findings include: (1) the properties of the cloned transporter are virtually identical to those of glucose transport across the brush border membrane; (2) specific SGLT1 antibodies recognize the protein in both immunohistochemistry and Western blots of brush border membranes; (3) inherited glucose galactose malabsorption (GGM) is due to defects in SGLT1 trafficking and/or function in the intestine; and (4) Sglt1 -null mice show defects in glucose absorption (see below).
Considerable progress has been made in our understanding of the mechanism of sodium-glucose cotransport by SGLT1, thanks to biophysical and biochemical studies in recombinant expression systems, success in solving the structure of the closely related bacterial sodium-galactose cotransporter vSGLT, homology modeling, and molecular dynamic simulations.
SGLT1 is a monomeric integral membrane protein of 72 kDa (without glycosylation) embedded in the lipid bilayer that normally couples the inward transport of two Na + ions and one glucose molecule across the membrane in each kinetic cycle. The system is completely reversible. The rate and direction of transport depends on the magnitude and direction of the driving forces, the sodium electrochemical potential gradient and the glucose concentrations outside and inside the cell. There is no glucose transport through SGLT1 in the absence of sodium but protons can drive cotransport. The maximum turnover rate is 1200 min − 1 at 37°C.
The crystal structure of the sodium/galactose symporter vSGLT from Vibrio parahaemolyticus revealed that the protein is composed of 14 transmembrane helices (TMs) with a core-inverted repeat of 10 TMs (TM1-5, TM6-10), and contains two discontinuous helices, TM1 and TM6 (see hSGLT1 homology model in Fig. 46.4 ). The core structure is virtually identical to that of the bacterial amino acid transporter LeuT and nine other cotransporters and exchangers in unrelated gene families. In all cases, the substrate-binding site is in the middle of the protein next to the discontinuous regions of TM1 and TM6. In the sodium cotransporters, one Na + -binding site (Na2) is formed by conserved serines and carbonyl oxygens on TM1 and TM8. There is a second Na + -binding site (Na1) in SGLT1, but the location of this site has not been definitively identified. However, mutation analysis indicates that the Na1 site overlaps with the sugar-binding site, as in the absence of sugar, mutation of residues implicated in glucose binding, namely H83, Y290, and W291 decreased the Na + affinity of the second site by > 10-fold.

The structure of vSGLT revealed that the glucose-binding site is in the center of the protein with gates isolating the bound sugar from the exterior and interior aqueous solutions on each side of the membrane (see homology model of hSGLT1 in Fig. 46.4 ). The first step in the transport cycle is the binding of external Na + to the Na2 and Na1 sites, which opens the external gate to permit external glucose binding ( Fig. 46.5 ). Closure of the external gate occludes the bound glucose from the external solution. The external vestibule and glucose-binding site are large enough to accommodate large (> 600 Å 3 ) glucopyranoside substrates such as indican and inhibitors such as phlorizin. Molecular dynamic simulations indicate that the sugar can rapidly exchange between the binding site and water in the large sugar pocket, before exiting to the cytoplasm through an aqueous vestibule. A combination of multiple molecular dynamic simulations and whole-cell patch clamp experiments show that sugar and Na + are released simultaneously into the cytoplasm before the protein completes the kinetic cycle. While the kinetic scheme for the inward cycle is ordered, that is, Na + binds before the sugar, the order of ligand binding in the reverse direction is not yet resolved. Na + /glucose cotransport generates a sodium current. The voltage-sensitive steps are the isomerization of the apo-protein in the membrane (transition between states 6 and 1 in Fig. 46.5 ) with a valence of 0.7, and external Na + binding (transitions between states 1 and 2) with a valence of 0.3. These voltage-sensitive steps are revealed as SGLT1 capacitive charge movements in the presence and absence of sodium. Both the substrate and the Na + on the Na2 site escape to the cytoplasm through the hydrophilic cavity formed by the cytoplasmic ends of TM1, TM2, TM5, TM7, and TM9 ( Fig. 46.4 ). Thus, a large aqueous vestibule extends from the external surface to the sugar-binding site in the outward-open conformation, and another large aqueous vestibule extends from the sugar-binding site to the cytoplasm in the inward-open conformation (states 2 and 4 in Fig. 46.5 ).

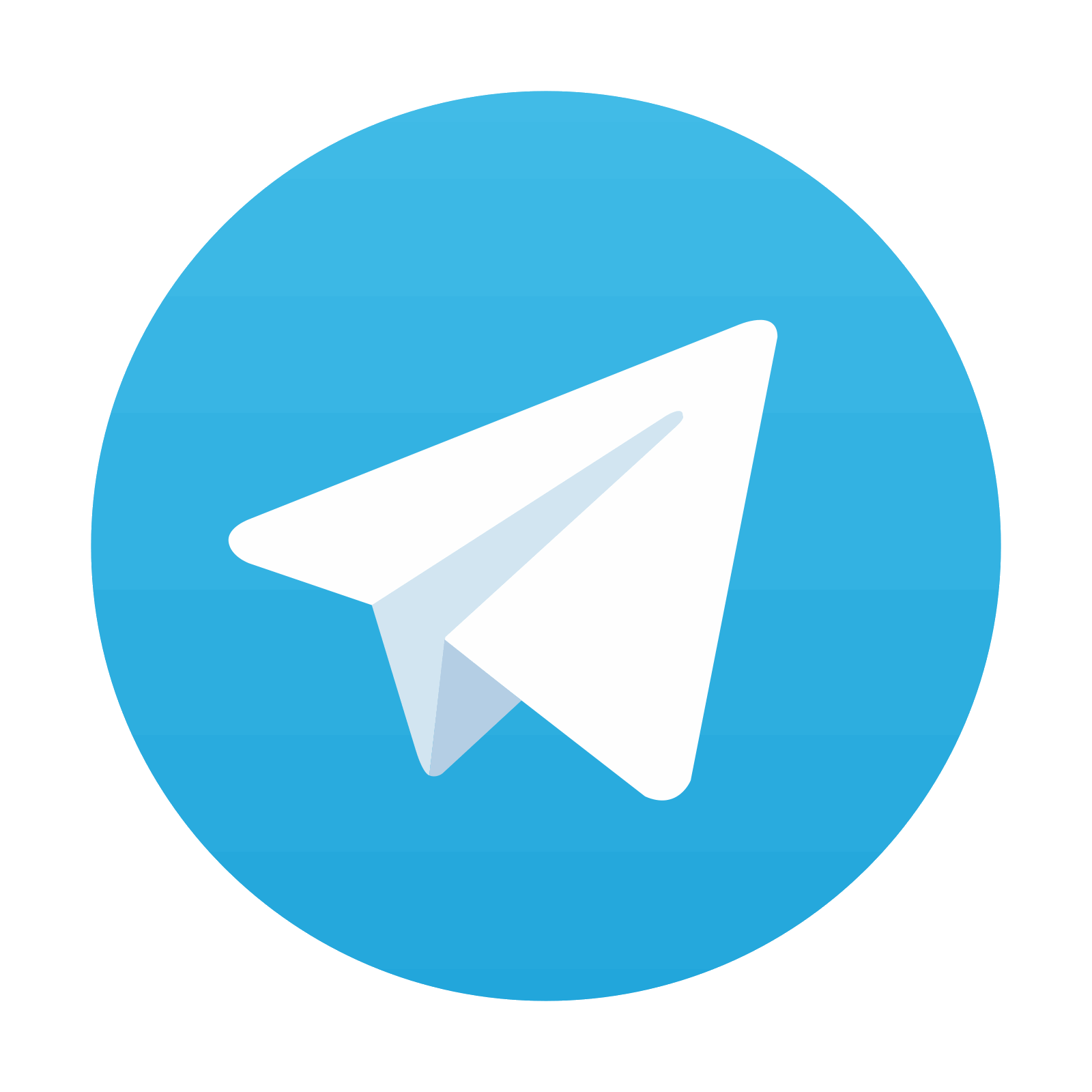
Stay updated, free articles. Join our Telegram channel
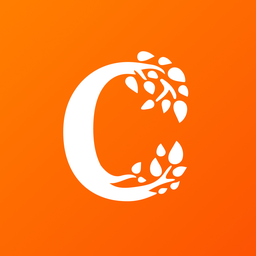
Full access? Get Clinical Tree
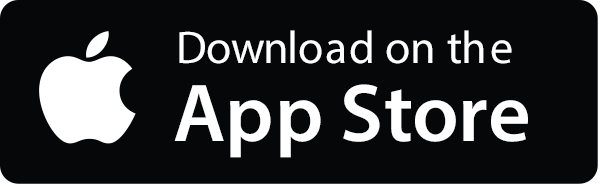
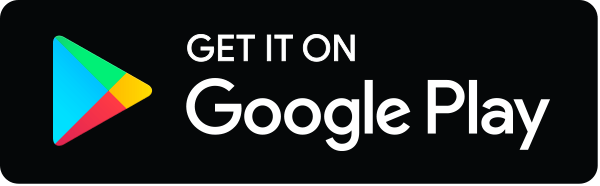
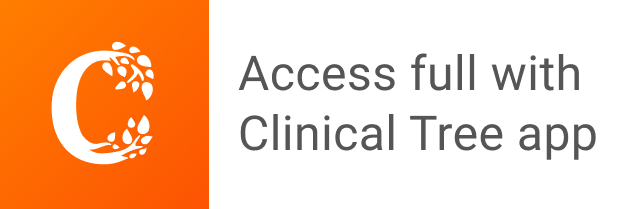