Abstract
The pancreatic acinar cell synthesizes, stores, and secretes the enzymes and enzyme precursors required for the digestion of dietary proteins, carbohydrates, and lipids. To meet the daily needs for digestion, the acinar cell has the highest average rates of protein synthesis in the body. Enzymes are stored in zymogen granules that are localized to the apical region of the cell. Signals generated by food in the intestine reach the acinar cell by neural and hormonal routes stimulate the secretion of enzymes from zymogen granules by exocytosis into the pancreatic duct. The acinar cell has been a model system for examining mechanisms of protein synthesis and export. Thus, nascent digestive enzyme proteins are transported from the endoplasmic reticulum to the Golgi complex, segregated from lysosomal enzymes, and then directed to zymogen granules by vesicular transport in a time-dependent and vectorial manner.
The exocrine pancreas has two major physiologic functions: it supplies the enzymes and enzyme precursors (zymogens) that are needed for digesting dietary lipids, carbohydrates, and proteins and secretes a bicarbonate-rich fluid that neutralizes acidic gastric secretions and thus provides the correct pH for intestinal digestion by pancreatic enzymes. Two major cell types form the exocrine pancreas; acinar cells and duct cells. The chapter focuses on the acinar cell which synthesizes, stores, and secretes digestive enzymes while duct cells secrete chloride and bicarbonate. Reasons to focus on the acinar cell include its critical physiologic function and its history as the model for the scientific studies that described the steps responsible for regulating protein synthesis and export and cell signaling. Thus, after electron microscopy was developed, cell biologists first visualized acinar cell organelles, they often then determined their cellular function by studying this cell. Although this review is largely based on the data from rodent acinar cells, limited data from human acinar cells suggest they will exhibit the same fundamental responses.
Keywords
Pancreas, Acinar cell, Protein synthesis, Vesicular trafficking, Exocytosis, Pancreatitis
Acknowledgments
Support for the investigative activities of FSG come from the NIH (DK 52401 and DK098108) and a Veterans Administration Merit Award and for SP DK098108. The authors also wish to thank Professor Guy Groblewski for reviewing sections of this chapter.
39.1
Organization of the Exocrine Pancreas
The pancreas is a retroperitoneal organ that is composed of the exocrine and endocrine glands. About 85% of the gland is exocrine and about 10% is extracellular matrix (ECM). While the duct cells and blood vessels comprise about 4% of the gland’s volume, the endocrine pancreas represents < 2% of pancreatic mass. The glandular portion of the pancreas often decreases in amount in diseases such as chronic pancreatitis or pancreatic cancer. The fraction represented by endocrine tissue can also vary depending on various pathologic conditions such as diabetes ( Figs. 39.1 and 39.2 ).


The fundamental structural units in the exocrine pancreas are macroscopically visible lobules. Each lobule is drained by interlobular ducts that combine to form larger interlobular ducts. These ducts gradually enlarge and coalesce to form the main pancreatic duct that delivers acinar and duct secretions into the duodenum.
The fundamental secretory unit of the exocrine pancreas is the acinus, a collection ~ 20–200 acinar cells, adjacent centroacinar cells which protrude into the lumen of the acinus, and proximal small ducts ( Fig. 39.2 ). The collective function of the cells in the acinus is to combine exocytosed secretory proteins from individual acini with water and electrolytes to form a mixture that can flow into the distal ductular system. In contrast to other glands such as the salivary glands, the exocrine pancreatic duct system does not always end in blind-ended lumen surrounded by acinar cells. Thus, wax-cast and microscopic reconstruction studies have shown that the most proximal ducts are often branched and interconnect with groups of acinar cells frequently forming a collar around the duct as well as the typical blind-ended gland structure ( Fig. 39.3 ; arrowhead in (B)—From Ref. ).

Although not a focus of this chapter, the exocrine pancreas are closely related to the endocrine gland. For example, as shown in a vascular wax-cast preparation of rat pancreas, portal system arises from the islet and supplies up to a half of pancreatic acinar cells ( Fig. 39.4 ). Similar relationships have been shown for human pancreas. The primary portal flow goes to acinar cell, with little if any going to duct cells in the human pancreas. Although this relationship exposes some acinar cells to much higher concentrations of islet hormones than found in circulation, the physiologic consequences of this relationship remain unclear. However, physiologically it could account for differences in acinar cell digestive enzyme content that depend on their proximity to islets. Pathologically, it could contribute to the pancreatic fibrosis that has been appreciated to accompany diabetes, especially that of the Type I form.

The close physical relationship between the endocrine and exocrine pancreas may be important in disease independent of the portal system. Thus, exocrine diseases including acute and chronic pancreatitis as well as pancreatic cancer can cause disorders of glucose metabolism through the effects these diseases have on function of islets of Langerhans. This type of diabetes is now called Type 3c diabetes and may result from both the loss of mass of the islets in the pancreas due to destructed associated with the disease process and mediators released from the diseased tissue that affects the function of cells with the islets of Langerhans. For example, there is a deficit in the secretion of the islet hormone pancreatic polypeptide that occurs with a meal in Type 3c diabetes and pancreatic polypeptide has been found to regulate the sensitivity to insulin in the liver. Thus, both the loss of islet cell mass and function occur in these exocrine pancreatic diseases and can result in disorders of glucose homeostasis.
39.2
Pancreatic Development
The exocrine pancreas arises from two anlagen of the primitive foregut: a dorsal portion coming from the dorsum of the duodenum, which forms a portion of the head and all of the body and tail; and a ventral portion derived from the primitive bile duct, which forms the remainder of the head and uncinate process. The ducts draining the dorsal and ventral pancreas fuse at about 6 weeks of gestation in humans, with the ventral duct providing the main conduit of drainage into the duodenum through the Ampulla of Vater. Postnatally, the different origins of the pancreas are reflected by the occasional persistence of separate dorsal and ventral ducts (known as pancreas divisum). In this condition, most pancreatic secretion enters the duodenum through the accessory pancreatic duct that arises from the dorsal pancreatic bud. Because the accessory duct is smaller than the major pancreatic duct, it can impede the flow of secretions and rarely cause an inflammatory disease of the pancreas known as pancreatitis . The arterial supply arises from branches of the splenic artery, which form arcades with the pancreatic branches of the gastroduodenal and superior mesenteric arteries. The autonomic innervation is both parasympathetic and sympathetic through splenic subdivisions of the celiac plexus.
As is the case for all endodermal glandular derivatives of the primitive gut endoderm, epithelial evagination is accompanied by the preservation of transepithelial integrity despite massive movements of epithelial sheets and rapid cell division as the epithelium expands, invades the adjacent mesenchyme, and differentiates. Pancreatic growth and differentiation is regulated by the sequential activation of specific genes. Critical for regulating the onset of pancreatogenesis is activation of the Pdx1 gene. A complex and not fully described series of signals from the mesenchyme, adjacent tissues, and the epithelium orchestrate the subsequent development of the endocrine and exocrine pancreas. The programs that mediate development of the dorsal and ventral pancreas are not identical. For example, in the earliest stages, the dorsal bud requires signals from the notochord and later from the aorta whereas ventral bud development requires signals that arise from the nearby developing liver and heart. Release of fibroblast growth factor and bone morphogenetic protein by the developing heart is required to drive differentiation of a portion of the ventral bud to form pancreas and not liver. Downstream of Pdx1 in pancreatic development is activation of NOTCH1 , which can also regulate the generation of acinar cells from centroacinar cells in adult pancreas. The latter function is important to reconstitute pancreatic function following pancreatic injury. Final differentiation of the exocrine pancreas requires the sequential activation of distinct genes for specific lineages. For example, Ptf1α followed by Mist1 activation and the interactions of these two factors in the pancreatic acinar are required for acinar cell specification and maintaining the acinar cell phenotype. Similarly, pancreatic innervation and vascularization requires the sequential activation of distinct genes. The importance of some of these developmental pathways, especially those that drive cell proliferation and transformation, is underscored by the potential roles in pancreatic tumorogenesis.
Despite the complex tubular-acinar structure in the mature gland, beginning with the cuboidal epithelial cells lining the opening of the main pancreatic duct at the sphincter of Oddi and continuing to the termination of the smallest duct/acinar structures, the epithelium maintains its continuity and is bounded by a basement membrane (BM), ensuring that the ductal or acinar luminal space maintains its physical separation from the interstitial space (see below and Fig. 39.5 ). It should be noted that intercalated duct cells mark the end of the acinar cell lumen and the beginning of the duct system. Intercalated duct cells are connected to each other and to adjacent acinar cells by junctional complexes that are typical of all epithelia (discussed below).

39.3
Structural Organization
39.3.1
Extracellular Matrix
Typical of all epithelia, acinar units and ducts of the pancreas are embedded in an ECM. All cells found in the ECM synthesize ECM molecules; ECM precursor or stem cells are derived from embryonic mesenchyme. Thus, the resident cells of the ECM, with the exception of transients such as phagocytic and immune cells, are capable of forming all of the cellular and molecular elements of the ECM.
The ECM has a number of functions including to organize and support the epithelium. Collagen and elastin fibrils provide tensile strength and resiliency, respectively, for the gland proper while reticular fibrils of Type III collagen form a meshwork that supports the parenchyma as it does in all glandular structures. The proteoglycans in which the structural elements of the gland’s ECM are embedded serve as a reservoir for water and electrolytes. The ECM binds cytokines and growth factors and so makes them available when needed. Tissue viscosity/stiffness is largely a function of the ECM and can signal to cells in the matrix as well as epithelial cells to modulate gene expression, tissue development, and differentiation. Mechanisms linking ECM signaling to the nucleus for regulating transcriptional activity have been described. Although the importance of tissue stiffness has been recognized in other tissues, such as the liver, it has not been studied in the pancreas, but should have important roles in the development, maintenance of the differentiated state, regeneration, and responses to fibrosis.
The ECM also acts as a physical barrier for infectious agents and is the site where phagocytic and immune cells play their roles during infections and inflammatory processes. A number of nonepithelial cell types including macrophages, stellate (Ito) cells, mast cells, and cells of the T and B lymphocytic line are embedded in the ECM. The formation of the majority of the ECM appears to be under the control of the stellate cell. In the resting state, prominent Vitamin A-filled vesicles in their cytoplasm can identify these cells. After activation, the Vitamin-A laden vesicles disappear and stellate cells proliferate and produce collagen. Such stimulation can occur during development, tissue repair, or regeneration. Some pathologic conditions, such as chronic pancreatitis and pancreatic cancers, are associated with persistent stellate cell activation and excess pancreatic fibrosis and positive feedback for proliferation between pancreatic cancer and stellate cells is described.
Integrating the ECM with the epithelial cells of the pancreas is the BM ( Fig. 39.5 ). The BM directly underlies the basal plasma membrane of the epithelium and is produced by the epithelial cells. It is composed of a cage of Type IV collagen in which is embedded a number of BM matrix components including negatively charged heparin sulfate proteoglycans and linker molecules such as laminin, nidogen, and perlecan. The linker molecule laminin has an important role in integrating the structure and function of the overlying epithelium with the ECM. Laminin is a multivalent molecule with binding sites for integrins on the basal plasma membrane of epithelial cells, proteoglycans, ECM collagen and the surfaces of ECM cells such as fibroblasts, adipocytes, and capillary endothelial cells. Because of these multivalent functions and the molecules to which it binds, the epithelium and the ECM are effectively a structural continuum.
The extensive functional interactions between epithelial cells and the ECM is often mediated by proteins of the integrin family. These transmembrane proteins are located in the basal plasma membranes of virtually all epithelial cells. In this location, they serve as receptors for various extracellular ligands, such as laminin, fibronectin, and collagens. Although they traditionally have been viewed as mechanical links between the overlying epithelium and the BM at focal contacts, and hence to the ECM, their function is much broader. By virtue of the fact that the cytoplasmic portion of most integrins are linked indirectly to the actin cytoskeleton and to organized signal arrays within the cell, integrins are able to transmit information from the extracellular environment into the cell. This information takes a variety of forms that are now being understood, including activation of signaling pathways for growth and differentiation and activation of transcription. Thus, signals from outside the cell arising in the external environment/ECM can affect cell function in the short term such as potentially changing the patterns of transcription of secretory proteins, and they may have long-term effects such as progression to neoplasia.
39.3.2
Intercellular Junctions
All cells in an epithelium, including the duct and acinar cells of the pancreas, are attached to each other by junctional complexes. These are functionally classified into three groups: (1) sealing or tight junctions; (2) adhering junctions, and (3) communicating or gap junctions. These structures are distinguished by their distribution, morphologic appearance, protein composition, and function.
39.3.2.1
Tight Junctions
Among the junctional complexes, sealing or tight junctions are always found closest to the lumen of glands and ducts and are classically described as providing an impermeable barrier to the intermixing of ions and other molecules between luminal contents and the interstitial space. As shown in Fig. 39.6 , transmission electron microscopy demonstrates that tight junctions are discrete structures within closely opposed regions of plasma membrane. Filamentous bands on the cytoplasmic side of tight junctions represent regions of attachment of cytoplasmic actin filaments. Freeze-fracture preparations that reveal the distribution of proteins within membranes show that tight junctions are composed of interdigitating bands that form a contiguous collar around the apical region of the cell ( Fig. 39.6 B).

Over a dozen proteins contribute to the tight junction including occludins, ZO1 and ZO2, and the claudins. The latter is a family of tetra-spanning membrane proteins, the claudins (from the Latin claudere “to close”). This family of proteins are the major determinant tight-junction permeability. Homophilic and heterophilic claudin complexes form strong adhesive structures between epithelial cells. Claudins are expressed in an epithelial-specific fashion; for example, claudin 16 is highly expressed in epithelial cells of the proximal tubules of the kidney, but little is found in other tissues. In the pancreas, claudin 2 is found only in junctions of the duct epithelia, claudin 5 is limited to junctions between acinar cells, whereas claudin 3 and claudin 4 are in both locations. Notably, tissue claudin expression shows plasticity that can change paracellular permeability in adult tissues.
Claudins are central to the structural functional basis of tight junctions and central to forming a highly selective barrier that both restricts the paracellular movement of large and small molecules and facilitates passage of certain ions between the lumen and the interstitial space. Ion selectivity of the paracellular pathway is determined by the charges of amino acids on the extracellular domain of tight-junction claudins. Claudin mutations are the cause of several human diseases including familial hypomagnesemia caused by mutations in claudin 16 (also known as paracellin-1) in distal tubules of the kidney. More recently, variants in claudin 2 have been found to be a risk factor for developing alcoholic pancreatitis as well as nonalcoholic pancreatitis, though the mechanism remains unclear.
ZO1 and ZO2 and possibly other proteins such as cingulin indirectly link claudins and possibly occludins to the actin cytoskeleton. Because of this association, it has been suggested that actin microfilaments, containing myosin II, may also be involved in the modulation of the paracellular pathway in response to signals. A variety of signaling molecules including cytokines can upregulate or downregulate paracellular permeability. These functions are effected by changing the levels of junctional proteins or by effecting their phosphorylation. For example, TGF-α increases permeability while EGF decreases permeability. The tight junction and other components of junctional complexes that forms an impermeant barrier ( Fig. 39.6 C) become disordered early in the course of experimental pancreatitis.
In addition to its central role in restricting the bidirectional movement of soluble molecules, tight junctions also help to establish and maintain acinar cell polarity by preventing the intermixing of membrane proteins and lipids between the apical and basolateral domains of epithelial cells by diffusion.
39.3.2.2
Adhering Zonules and Desmosomes
All cells in an epithelium are mechanically connected to each other through two types of junctions, both of which use homotypic interactions between cadherins expressed and localized in neighboring cells. These are adhering zonules which form belt-like arrays of adhering junctions immediately beneath tight junctions in all epithelial cells, and desmosomes which form punctuate adhering zones between cells of epithelia subject to mechanical shear. The cytoplasmic portion of cadherins in adhering zonules is associated with anchoring proteins that in turn interact with cytoplasmic actin filaments. It is believed that the contraction of actin filaments in the adhering zonule belt results in a purse string constriction of the apices of some epithelial cells thereby causing the epithelium to evaginate as it occurs during gland morphogenesis. Desmosomes, on the other hand, have their cytoplasmic anchor proteins associated with intermediate filaments. Since intermediate filaments do not possess plus and minus ends as do actin and microtubules, the filaments associated with desmosomes do not have contractile function and serve primarily to resist mechanical shear among cells in an epithelium. All epithelial cells are also associated with their underlying BM through hemidesmosomes whose cytoplasmic cytoskeletal attachments are also coupled to intermediate filaments but whose transmembrane linker proteins are members of the integrin family of transmembrane proteins. As discussed above, integrins interact with ligands in the BM, one of which is laminin, a multilinker glycoprotein that serves to integrate the BM with cells and molecules of the adjacent ECM.
39.3.2.3
Gap Junctions
Epithelial cells are functionally connected to each other through gap junctions that allow direct communication between the cytoplasm of adjacent acinar cells ( Fig. 39.7 ). A distinct protein family known as connexins assembles in hexamers to form small transmembrane pores between cells known as connexons. Large rounded groupings of connexons forming gap junctions are found in the lateral membranes of adjacent cells. The connexon pores demonstrate size and ionic selectivity and do not allow molecules with molecular weights > 1000 to pass. Their main physiological functions are to coordinate signaling between cells by allowing the movement of signaling molecules such as calcium, inositol trisphosphate (IP3), and cAMP through the junction. Thus, hormonal signals impinging on one or a few cells in an acinus are coupled to synchronize the exocytosis of zymogen granules. The channels also close rapidly in response to intracellular acidification or high levels of cytosolic calcium, preventing spread of damage between cells in a wounded epithelium.

The importance of acinar cell gap junctions has been examined using chemical inhibitors and mutant mice that are deficient in specific connexins and studied in the pancreas and other tissues. Several generalizations can be drawn from these studies. First, pancreatic acinar cells are coupled by gap junctions containing connexins 26 and 32 that allow molecular messengers, including calcium and other signaling molecules, to spread from one cell to the next. In mice lacking connexin 32 the number of gaps junctions is greatly reduced and intercellular communication assessed using a transferable dye is inhibited. Interestingly, genetic absence of connexin 32 caused increased pancreatic enzyme secretion, but electrical coupling was maintained. These findings suggest that gap junctions might suppress pancreatic secretion, but electrical coupling can be mediated by gap junctions formed only by connexin 26. Notably, the connexin 32 −/− mice exhibited a much more severe form of caerulein [a cholecystokinin (CCK) analogue] hyperstimulation pancreatitis than wild-type controls. These studies suggest that the overall effect of the gap junctions formed by connexin 32 in pancreatic acinar cells is to dampen both basal secretion and the pancreatitis response to hyperstimulation. Although intracellular pH and calcium levels can affect gap-junction function, the extracellular environment can also affect the biogenesis of this organelle. Thus, reducing extracellular pH affects the turnover rate of acinar cell connexon-32 and gap-junction coupling. Such a response could worsen pancreatitis responses if blood flow is reduced, causing tissue acidosis, during acute pancreatitis. Finally, an unexplored contribution to pancreatic injury and inflammation may come from hemi-channels formed by connexons or a related family of protein known as pannexins. The hemi-channels are plasma membrane pores that are not connected to opposing cells. Although normally closed, they could open under pathologic conditions releasing cellular ATP into the interstitium and stimulate innate immunity.
39.4
Functional Responses of the Acinar Cell: Protein Synthesis, Vectorial Transport, Modifications, and Sorting
The exocrine pancreas has the highest daily rates of protein synthesis of any organ in the body. Thus, 1000–1500 mL of pancreatic exocrine secretion with 10–100 g/L of protein reaches the small intestine each day. The pancreatic acinar cell is elegantly designed to meet the requirements for both high levels of protein synthesis and the storage and release of secretory proteins. These distinctive features have made the pancreatic acinar cell a key system for biologic studies. Indeed, the intracellular itinerary of nascent secretory proteins was first described in the pancreatic acinar cell. Synthesis and intracellular movement of export proteins is an ordered and sequential process that involves the vectorial transport of proteins through specific organelles. It begins with nascent protein synthesis in the endoplasmic reticulum (ER), vesicular transport of nascent proteins to the Golgi complex, their movement to zymogen granules, and finally secretion from the zymogen granule storage compartment by regulated exocytosis. The major organelles responsible for protein synthesis, transport, and storage are shown in Figs. 39.8–39.11 . After synthesis, newly formed proteins undergo covalent modifications and assume their tertiary structure with the aid of chaperone proteins that facilitate and monitor correct folding, a process termed quality control . As nascent secretory proteins undergo vectorial anterograde transport, they are concentrated and are segregated away from nonsecretory proteins, such as proteins that will reside in lysosomes and newly synthesized membranes proteins. This review will emphasize mechanisms relevant to soluble secretory proteins responsible for nutrient digestion in the intestinal lumen.




39.4.1
Protein Synthesis
The synthesis of new secretory proteins occurs on and in the ER. Two ER forms can be distinguished morphologically and have distinct functions: the rough ER (RER) and smooth ER (SER). The RER can be recognized by the ribosomes on its cytoplasmic surface, while the SER lacks ribosomes ( Figs. 39.8 and 39.9 ). Specialized regions that are contiguous with the RER and SER have been termed transitional elements. The ER exit sites, locations in the transitional ER, a specialized domain in which both the membrane and soluble proteins are transported from the ER in COPII coated vesicles (see Section 39.4.4 ). The RER, transitional elements, and SER enclose ( Fig. 39.9 ) a contiguous lumen known as the intracisternal space. Within this space, nascent export proteins, such as those destined for zymogen granules and the lysosome, begin their folding and covalent modifications as described below (see Section 39.4.3 ). The acinar cell has an extensive RER to meet its requirements for high levels of secretory protein synthesis. Although the RER is most evident at the base of the acinar cell, it extends up to the apical region of the cell ( Figs. 39.1 and 39.8 ). The width of the RER cisterna ranges from 20 to 80 nm and enlarges during active protein synthesis; all cisternae appear to be contiguous. Sites of active protein synthesis are marked by regularly placed rosettes of electron dense ~ 30 nm ribosomes that are attached to the cytosolic face of the ER membrane ( Fig. 39.8 ). These represent areas active in translation and are ribosomes associated with messenger RNA (mRNA).
Morphologic and functional studies provide evidence that there are mechanisms for regional specialization within the ER. For example, in some regions of the ER ribosomes are attached to the cytoplasmic face at regular intervals (RER) while other regions of the ER are devoid of ribosomes. Functional specialization of the ER is also demonstrated by calcium signaling. Thus, the initial increase in cytosolic calcium in the acinar cell occurs at the apex of the cell and represents release from specific regions of the ER. This particular phenomenon results from the localization of the IP3 calcium channel to the SER at the apical-most region of the acinar cell (see below). Distinct structural links between the ER and other membrane compartments have been observed by electron microscopy, but insight into how these contacts are formed and their physiologic functions has only recently been described. Contacts between the ER and mitochondria and ER and plasma membrane are among those that have been described. For example, pancreatic acinar cell calcium homeostasis involving the apical region of the cell revolves around distinct apically distributed IP3 receptors (ER) that release calcium into the cytosol and closely approximated regulators of calcium entry into the ER (Orai1, STIM, and others) that are activated when depletion of ER calcium is sensed. Specific proteins, such as extended synaptotagmin I, are required to establish these specialized membrane domains that bring the ER and plasma membrane into close stable proximity. Membrane contacts, similar to those that tether the ER and mitochondria and that facilitate the exchange of glycerol lipids between opposed membranes, are likely to participate in content exchange between other tethered cellular organelles.
The ribosome is formed by large and small subunits of the ribosome macromolecular complexes of proteins and specific ribosomal RNA; the large and small subunit can be distinguished by transmission electron microscopy. New protein synthesis takes place in a groove that lies between the two subunits and is directed by mRNA. Specific groups of soluble proteins regulate the initiation, elongation, and termination phases of protein synthesis. The first synthesized component of a secretory protein is a specific amino-terminal extension known as the signal sequence. The signal sequence is 15–50 amino-acid peptides that contains a hydrophobic core. Also known as a leader sequence , the signal sequence allows nascent proteins to enter the ER through a macromolecular multiprotein channel known as the translocon. This signal sequence binds to a signal recognition particle (SRP) that directs the connection of the early nascent protein and mRNA to the ER by attachment to its receptor, SRP receptor. After attachment, the SRP is released and the signal peptide enters the ER protein-conducting channel. As a nascent secretory protein enters the ER, the signal sequence is removed by a resident protease, trapping the protein in the ER lumen. The translocon also mediates the insertion of transmembrane proteins into the ER membrane and has a role in protein degradation (discussed elsewhere). In addition to its critical role in the initial steps of protein synthesis, the ER has other specialized functions that include cell signaling, protein folding, and specific protein modifications.
39.4.2
Vectorial Movement of Proteins
The sequential movement of nascent proteins between secretory compartments was first demonstrated using the pancreatic acinar cell and pulse-chase protocols. In this type of study, isolated cells or tissues were transiently incubated with tracer amounts of a radioactive amino acid and then this was washed away and replaced with unlabeled amino acids. Nascent proteins incorporate the pulse of radioactive amino acids and can be followed as they traverse the cell. The labeled proteins can be detected using autoradiography or by assaying isolated subcellular fractions for radioactive amino acids incorporated into proteins ( Fig. 39.12 ). This approach demonstrated that nascent proteins moved through the acinar cell in a vectorial manner over time from their site of synthesis in the ER to be stored in zymogen granules. The classic studies demonstrating the stepwise movement of new secretory proteins used a pulse of tracer amounts of a radioactive amino acid followed by a large excess of the nonradioactive form of the amino acid to label nascent protein. The itinerary of the newly synthesized radio-labeled protein was tracked over time using electron microscopy with autoradiography ( Fig. 39.12 ) as well as cell-fractionation studies. These studies showed that nascent proteins quickly left the ER to reside in the Golgi complex, then moved from the Golgi complex to condensing vacuoles (CV) [immature secretory granules (ISGs)], and finally to zymogen granules. Note that it takes almost 60 min for the first nascent protein to reach zymogen granules. Subsequent studies have examined the conditions for nascent proteins entering the ER, the regulation of vesicular movement between compartments, and the distinct protein modifications that occur in specific organelles and are summarized below.

Nascent secretory proteins are subject to a variety of modifications; many of these occur within specific cellular organelles. Protein modifications often require the activity of accessory resident proteins that are concentrated in specific organelles. The mechanisms that restrict resident proteins to specific organelles are not fully understood. However, many soluble resident ER proteins contain the amino acid sequence KDEL or a similar sequence that binds the ER resident KDEL receptor, a seven-transmembrane domain protein. While the KDEL receptor and KDEL proteins can escape from the ER and travel to the Golgi, they are efficiently retrieved using retrograde vesicular transport back to the ER. Similarly, a KKxx cytoplasmic sequence that binds to COPI proteins enables ER membrane proteins to return to the ER from the Golgi complex. The KDEL receptor has a structure similar to that of a heterotrimeric G-protein and may function to regulate both antegrade and retrograde movement of KDEL containing proteins by interacting with distinct signaling pathways.
39.4.3
Protein Modifications
39.4.3.1
Cleavage of the Signal Peptide, Folding, and Covalent Modifications
One of the first modifications of secretory proteins within the ER is the proteolytic removal of the signal peptide. The cleavage is mediated by a specific ER protease (signal peptidase) and occurs within the translocon after a large portion of the nascent protein has entered the ER cisterna. Removal of the signal peptide traps the nascent protein in the secretory pathway unless the protein misfolds. Protein folding is a critical ER event that requires the coordinated and iterative interactions of a number of accessory proteins. The ER resident proteins such as calnexin, HSP70, calreticulin, and Bip participate in this process. Calnexin is a calcium-dependent lectin that anchors nascent proteins in the ER, while HSP70 and other accessory proteins directly folds nascent proteins. The attachment of nascent glycoproteins to calnexin during the folding process is mediated through reiterative process that involves addition of a carbohydrate residue and its removal. The distribution of calnexin in the ER can vary and is concentrated in the regions of RER as well as near regions of the SER that abuts mitochondria . Although this glycosylation cycle is required for the folding of carbohydrate-labeled proteins, the mechanism responsible for the quality control of nonglycosylated proteins, such as host proteins destined for export by the acinar cell, remains unclear.
Proper protein folding and development of tertiary protein structure often requires covalent and noncovalent modifications within the ER. These include glycosylation, proline hydroxylation, and disulfide bond formation. The latter is regulated by protein disulfide isomerase activity. Secretory proteins are rich in disulfide bonds because they have a greater need for protection from an oxidative environment when they exit the cell, in contrast to cytosolic proteins, which reside in a reducing environment. Specialized protein modifications also occur in the Golgi complex and include modification of carbohydrate residues, sialylation, sulfation, and addition of mannose 6-phosphate (M6P) residues to lysosomal enzymes.
39.4.3.2
Protein Misfolding and Degradation
Proteins that are not properly folded are detected by an ER quality control system. Most misfolded membrane proteins are immediately degraded by a cytoplasmic multiprotein complex known as the proteasome. Since misfolded proteins formed within the ER are degraded in the cytoplasm, they must cross the ER membrane. This is accomplished by their retrograde transport through the protein channels in the ER such as the translocon. When a misfolded membrane or export protein begins to emerge retrograde into the cytosol through the ER protein channel, multiple copies of a small protein known as ubiquitin are attached. This modification is an address marker that directs the misfolded nascent protein into the ER-associated degradation (ERAD) pathway. The attachment of poly-ubiquitins guides misfolded proteins into a multiprotein complex known as the proteasome. The proteasome is a large (> 1 × 10 6 Da) macromolecular protein complex that has a cylindrical structure with a cap on one end to regulate protein entry. Access of ubiquitinated proteins to the core of the proteasome is ATP dependent. Multiple classes of proteases within this core degrade ubiquitinated proteins to small fragments that can easily be metabolically recycled for use within the cell. The proteasome is present in soluble and membrane-associated forms. The ER membrane-associated proteasomes appear to be located in close proximity to the translocon. Since misfolded nascent proteins emerge at the ER translocon, such a distribution would make degradation of misfolded proteins efficient. Misfolded membrane and soluble proteins can follow slightly different itineraries. Although misfolded membrane proteins are immediately degraded, at least some soluble proteins must transit to the Golgi complex and return to the ER for degradation by the proteasome.
39.4.3.3
ER Stress and the Unfolded Protein Response
The first steps cells take in response to misfolded proteins include reducing overall protein synthesis and increasing the levels of cofactors that mediate protein folding and export. If persistent, unfolded proteins are directed in degradation pathways. However, sometimes these responses are overwhelmed and unfolded proteins accumulate. Accumulation of unfolded proteins leads to the activation of a complex feedback mechanism known as the ER stress response. This response is characterized by a complex molecular hierarchy that begins with steps to correct protein misfolding, but can progress to activation of cell death pathways if unresolved. The first step in this response is to block general protein synthesis while increasing the synthesis of proteins involved in ER folding and export. When this measure is not successful and the levels of unfolded proteins continue to increase, additional components of the ER stress response are activated. These can lead to apoptotic or programmed cell death. There is increasing evidence on the role of the mechanisms of ER stress in protecting the pancreatic acinar cell from injury as well as participating in the mechanisms of pancreatitis. An example of protection is the ability of the acinar cell to use ER stress pathways to increase the folding and exporting machinery to withstand the protein folding disorders that take place with alcohol intake which increases incorrect disulfide bond formation and misfolding in the ER. When this protective ER stress mechanism is prevented, acinar cell injury and pancreatitis can follow. This protective mechanism likely accounts for the fact that pancreatitis only occurs rarely in people who drink excessive amounts of alcohol.
Pathologic ER stress responses leading to cell death can occur in several important disease states. For example, Hereditary Pancreatitis is caused by various mutations in cationic trypsinogen. A few mutations appear to cause disease by producing misfolded trypsinogen and a damaging ER stress response. Likewise, protein misfolding is central to the pathogenesis of the most common form of cystic fibrosis, the D508 mutation in the cystic fibrosis transmembrane conductance regulator (CFTR). Such misfolding could contribute to the appearance of pancreatic disease in individuals with cystic fibrosis.
39.4.4
Sorting and Concentration of Nascent Proteins
39.4.4.1
ER to Golgi
Secretory proteins are efficiently stored at high concentrations in zymogen granules by steps that sequentially enrich the proteins several hundred-fold over that found in the ER lumen ( Fig. 39.13 ). They are also segregated away from other soluble proteins, most notably lysosomal enzymes, as they move to their final storage site. The first step in the concentration process takes place as nascent secretory proteins are preparing to be transported in vesicles from the ER. These vesicles emerge from distinct protrusions from the RER, known as transitional elements, that are devoid of ribosomes and are close to the Golgi complex ( Fig. 39.9 ). After budding, the small (~ 90 nm) vesicles with their nascent protein cargo move to an intermediate compartment known as the vesicular-tubular complex (VTC). Proteins are then transferred from the VTC to the Golgi complex by direct fusion or by vesicles that bud from the VTC.

Movement of nascent proteins from the ER is mediated by the addition of the COPII coatamer protein complex to the cytoplasmic side of the ER membrane. The COPII coat proteins are required for cargo selection, vesicle budding from the ER, and forward trafficking. The coat is sequentially assembled with the addition of the small GTPase, Sar1, followed by the soluble complexes of Sec23/24 and Sec13/31. Accessory proteins may function as a scaffold for assembly and regulate the GTPase activity of Sar1. The mechanisms for cargo selection are not fully understood (see Ref. ). Some studies suggest that putative cargo may be concentrated into a molecular scaffold at the transitional elements of the ER. The selection of some transmembrane proteins for cargo appears to largely take place through the binding of distinct sequences on their cytoplasmic domain to Sec24 (reviewed in Ref. ). The COPII proteins are concentrated on the forming vesicles and transmembrane proteins that bind Sec24 are also enriched. Transmembrane proteins are enriched 5- to 10-fold in the COPII vesicle as compared to the nearby ER.
The mechanism for concentrating soluble proteins remains uncertain, but several findings demonstrate that it must be different than transmembrane proteins. First, while transmembrane proteins are greatly concentrated as they emerge in COPII vesicles, soluble proteins do not become substantially concentrated over the ER lumen until they reach the VTC. For the acinar cell, dramatic differences among concentrating secretory proteins emerging from the ER have been observed ( Fig. 39.13 ). Second, mechanisms described for concentrating soluble glycoproteins in other systems are only relevant to a limited number of pancreatic proteins. Thus, the carbohydrate moiety on some soluble glycosylated proteins binds to the lectin domain on the luminal domain of the transmembrane protein, ERGIC-58. It is possible that glycosylated pancreatic secretory protein such as GP2 and muclin are concentrated as they leave the ER by binding to ERGIC-58. However, other mechanisms must account for the concentration of most secretory proteins observed in the acinar cell. As shown in Fig. 39.13 , concentration of acinar cell secretory proteins is not uniform, with differences of over an order of magnitude being observed for amylase, chymotrypsin, and procarboxypeptidase A, and occurs at multiple compartments in the secretory pathway. It is possible that zymogen granule membrane proteins may participate in the concentration of exportable proteins. The net effect of the enrichment mechanism is to concentrate soluble proteins such as amylase, trypsinogen, and chymotrypsinogen 10- to 20-fold between the ER lumen and the Golgi complex and even further enrichment as they move toward the zymogen granule.
39.4.4.2
Golgi Complex to Trans-Golgi Network
The Golgi complex receives nascent proteins that have been carried from the ER in vesicles. Multiple membrane stacks, transport vesicles, and the trans-Golgi network (TGN) form the Golgi complex. The cis-Golgi is closest to the nucleus while the trans-Golgi is the most distal. Unlike the ER, the cisternae of the Golgi stacks are not contiguous and their widths are not uniform. Thus, the central area of a Golgi stack is flattened and the lateral region is enlarged. The lateral enlargement is likely required to accommodate the vesicular traffic in this domain. Some resident Golgi proteins such as membrane-associated, membrane, and luminal are concentrated within distinct domains. For example, the structural proteins GRASP 65 and GM130 are restricted to the cis-Golgi while GRASP 55 localizes to the medial Golgi and Golgin97 to the trans-Golgi. Collectively known as Golgins, these proteins help establish the spatial relationships among Golgi compartments and with adjacent compartments, participate in both vesicular tethering prior to fusion and tubulovesicular budding, and mediate microtubule attachments. GRASP65 and GRASP55 are cytoplasmic facing Golgi proteins that link the Golgi ribbons into stacks. Within the Golgi stacks, resident luminal facing proteins are restricting Golgi to distinct domains by mechanisms that remain unclear. However, the selective distributions of Golgi enzymes reflect their specific and sequential roles in protein processing (reviewed in in Ref. ). The Golgi is anchored in position by microtubules and the minus-end motor, dynein. Small vesicles (~ 100 nm) transport proteins in an anterograde and retrograde manner between the Golgi stacks. Thus, while secretory proteins are being moved anterograde, ER and Golgi resident proteins undergo retrograde transport and the ER and Golgi are returned to their proper localtion. Retrograde protein transport from the Golgi complex to the ER as well as within the Golgi complex requires COPI coat proteins; COPI proteins may also mediate some antegrade traffic in the Golgi complex. The TGN is formed by tubules and vesicles that arise from the trans-Golgi stacks. However, the TGN is biochemically distinct from the Golgi complex. For example, proteins such as TGN-38 are much more concentrated in the TGN compared to the cis and medial Golgi.
Specific protein modifications take place in the Golgi complex and TGN and are catalyzed by resident proteins. Thus, terminal carbohydrate residues are modified, M6P residues are attached to lysosomal enzymes, and proteins are sialylated and sulfated. The pancreatic acinar generates few O-linked glycoproteins; this modification appears to take place exclusively in the Golgi complex.
A gradient of decreasing pH is found in the Golgi complex and TGN. Thus, the pH within the Golgi stacks is estimated to be just below 7.0, while within the TGN it may be as low as 6.0. The low pH in the TGN may have a role in protein sorting. Thus, sulfation of glycoproteins and a low-pH environment may be required for sorting digestive enzymes to the zymogen granule. A possible target for this pH-dependent modification may be zymogen granule transmembrane proteins with a luminal glycosylated domain. Preliminary studies suggest that muclin may bind specific secretory proteins in a pH-dependent manner and that its absence affects secretory protein trafficking.
One of the best-characterized protein sorting mechanisms, for lysosomal enzymes, occurs in the Golgi complex. The M6P receptors on the Golgi complex recognize the M6P address marker on lysosomal enzymes. After binding its lysosomal enzyme cargo, M6P receptors are concentrated in clathrin-coated vesicles. The subsequent fusion of these vesicles with endosomes results in the acidification of the vesicle and dissociation of the lysosomal enzyme from the receptor. The lysosomal enzyme is then transported by an endosomal carrier to the lysosome. The M6P receptor then recycles back to the Golgi complex. That lysosomal enzymes lacking M6P modification can sort to the acinar cell lysosome as recognized. The M6P receptor-independent mechanisms for the delivery of lysosomal enzymes, targeted through LIPM-2 and sortilin have been described in other systems, but have not been studied in the acinar cell.
39.4.4.3
TGN to Zymogen Granules
The TGN is a major setting for protein sorting. In addition to directing lysosomal enzymes to lysosomes, budding vesicles that form the constitutive (unregulated) pathway, and developing zymogen granules arise from this compartment. Distinct vesicular carriers with morphologically and functionally different coats or lacking coats are found budding from the TGN and reflect the varying cargo that emerges from this compartment and are targeted to specific organelles ( Fig. 39.11 ). Studies of the TGN in living cells have shown that it is a dynamic structure with tubules protruding and retracting along microtubules. The expanded terminal regions of the TGN form precursors to zymogen granules known as CV or ISG. These structures are larger than mature zymogen granules and their content is less electron dense ( Fig. 39.10 ).
Maturation of CV to zymogen granules involves a reduction in the amount of membrane by budding of small vesicles. Some studies have suggested that vesicles formed from CV comprise those found in the constitutive-like (CL) or minor secretory pathway described elsewhere. The presence of M6P receptors on vesicles budding from CV likely provide a mechanism for retrieving lysosomal enzymes that have been sorted into the regulated secretory pathway. Whether these vesicles return lysosomal enzymes to the Golgi complex or other cellular compartment or direct them to the cell surface for secretion is not known. The lysosomal membrane protein, LAMP1, also traffics through CV and the minor secretory pathway before traveling to its final destination in the lysosome.
The mature zymogen granule has several important features including concentration of secretory proteins, as reflected by its characteristic electron-dense appearance when viewed by electron microscopy ( Fig. 39.10 ) and its association with proteins that affect its function. The formation of zymogen granules including packing of a large number of proteins is facilitated by a low pH and sulfated glycoproteins. The glycoproteins segregate to the inner surface of the granule membrane where ionic and hydrophobic interactions promote accumulation and aggregation of secretory proteins. These conditions allow for the accumulation of a large number of secretory proteins without increases in osmotic force, preventing them from rupturing. Advanced techniques to enrich organelles and identify resident proteins have identified resident and associated proteins of the zymogen granule. Although the function of many of the membrane proteins remain unclear and will guide future studies, the function of some such as the t-SNARES VAMP2 and VAMP8 have been better defined.
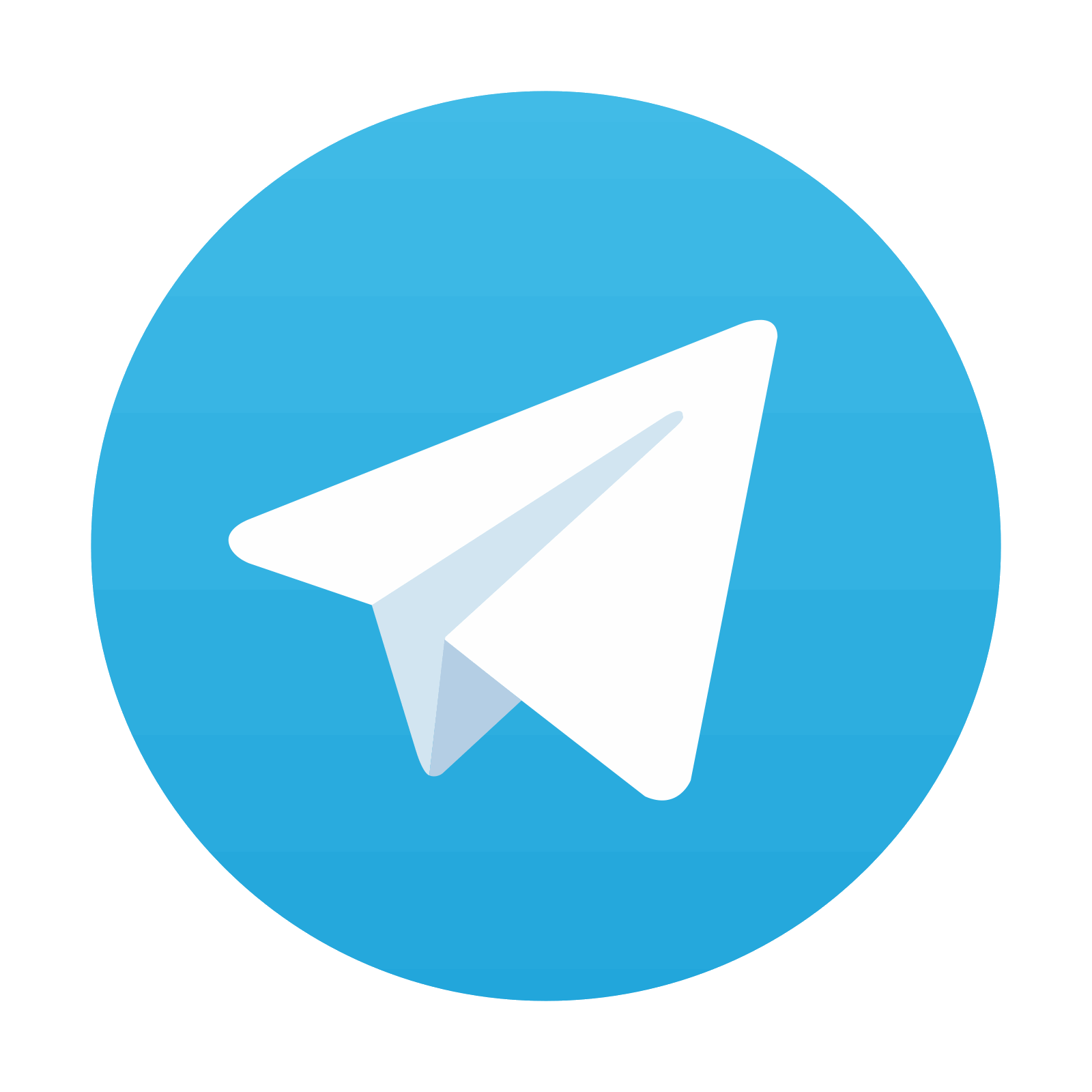
Stay updated, free articles. Join our Telegram channel
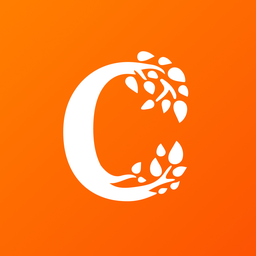
Full access? Get Clinical Tree
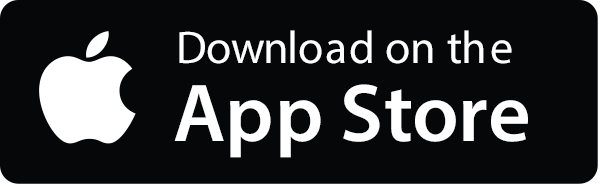
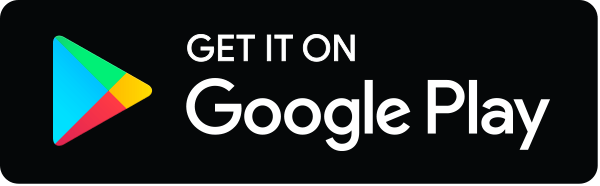