Abstract
Saliva coats the surfaces of the mouth, providing a protective, lubricious layer that maintains homeostasis of the oral environment. Salivary glands in humans and other mammals consist of three paired “major” glands, parotid, submandibular, and sublingual, which contribute 90% or more of the total volume of saliva in the mouth. There are also, many “minor” submucosal salivary glands; in man, many hundreds of these glands secrete a mucinous saliva onto the overlying mucosa. The structure of major salivary glands is a branching ductal tree terminating in acinar secretory units, which form saliva that is conducted through the ductal tree and delivered to the oral cavity. Production of saliva is almost entirely dependent on a nerve-mediated reflex initiated by taste, chemo- and mechanoreceptors associated with oral structures. Impulses in the afferent arm of the nerve reflex reach salivary centers in the brain and here impulse traffic is influenced by other centers in the brain before efferent autonomic nerves deliver impulses to salivary glands. Impulse traffic and saliva production is increased on food consumption, facilitating the eating and tasting of food. Salivary acinar cells produce saliva predominantly through intracellular calcium signaling following activation of muscarinic receptors. Salivary acinar cells comprise a salt and water secreting epithelium, requiring key membrane transporters and underpinned by an ionic gradient created by sodium potassium ATPase; the saliva secreted is isotonic. Acinar cell calcium signaling is susceptible to disruption by inflammatory stimuli leading to reduced salivary secretion. Acinar cells are also specialized for synthesis, storage, and delivery of large amounts of secretory proteins, including mucins, amylase, and many other functionally important proteins. The salivary ductal cell epithelium is salt absorbing, producing a hypotonic saliva, which is delivered to the mouth. The composition of salivas secreted by salivary glands, in particular the ionic and protein components enable the formation of viscoelastic fluid films and more adherent protein layers on oral surfaces, which are crucial to fulfillment of a number of important functions and maintenance of oral homeostasis. Major reductions in the coating of oral surfaces can result from salivary gland dysfunction and are associated with oral and systemic disease, which can affect severely on the quality of life.
Keywords
Saliva, Salivary gland, Secretion, Nerve reflex, Autonomic, Cholinergic, Calcium signaling, Salivary protein, Mucin, Salivary film
37.1
Introduction
Saliva is produced by salivary glands in a range of animal species and fulfills many different functions. Of particular importance are its roles in nutrition, facilitating the chewing, tasting, digestion, and swallowing of food. Striking dietary related adaptations in salivary gland structure and physiology are seen in mammals, reptiles, and invertebrates. For example, the production of venom in order to immobilize prey has evolved in different species of gastropod, mammal, and reptiles while female anopheles mosquitos ( Anopheles albimanus ) produce a saliva containing anophelin, a thrombin inhibiting anticoagulant similar to anticoagulants produced by snake venom glands. There is an immediate, increased demand for saliva in relation to food consumption, mediated by sensory stimulation, and a nerve-mediated reflex. Large volumes of saliva are secreted by sheep salivary glands and enter the rumen in order to facilitate digestion and fermentation of a cellulose-rich diet while the giraffe produces richly mucinous saliva that lubricates and facilitates the consumption of a diet rich in leaves of acacia thorn trees. The paired major salivary glands, parotid (PG), submandibular (SMG), and sublingual (SLG), can increase their production of saliva in response to stimulation.
Apart from the immediate needs relating to diet saliva coats the surfaces of the mouth, providing a protective, lubricious layer that maintains homeostasis of the oral environment. Major reductions in levels of saliva in the mouth are therefore associated with oral and systemic disease and can impact severely on quality of life. The oral environment is a balanced ecosystem in which communities of microorganisms (microbiome) form biofilms on saliva-coated surfaces. In addition to the major salivary glands hundreds of small, minor submucosal salivary glands, exocrine glands similar to those underlying other mucosal epithelia in the upper respiratory and gastrointestinal tracts play an important role in fluid film formation on oral mucosal surfaces. Although its contribution to whole mouth saliva is < 10% by volume, saliva from minor salivary glands is a lubricant for the oral mucosa, owing to its high mucin content.
37.2
Salivary Gland Anatomy and Structure
Salivary glands in humans and other mammals can be classified according to size into three paired major glands, PG, SMG, and SLG, which contribute to 90% or more of whole mouth saliva and variable number of glands (600–1000 in humans) forming minor structures located in the labial, palatine, buccal, lingual, and sublingual submucosae.
37.2.1
Anatomy of Major Glands
Major salivary glands consist of branching ductal trees terminating in secretory units referred to as acini; saliva formed by acinar cells is conducted through the ductal tree to be delivered to the oral cavity. In clinical investigations, this arrangement is well demonstrated by sialography when X-ray contrast medium is introduced retrogradely into the ductal tree ( Fig. 37.1 ). The paired PGs are the largest of the human salivary glands, weighing approximately 15–30 g and located in the preauricular region and along the posterior surface of the mandible ( Fig. 37.1 ). Each PG is divided by the facial nerve into a superficial lobe, lateral to the facial nerve, and overlying the lateral surface of the masseter and a deep lobe medial to the facial nerve and located between the mastoid process of the temporal bone and the ramus of the mandible. An accessory PG that may contain mucinous acinar cells in addition to the serous acinar cells can be found lying anteriorly over the masseter muscle between the parotid duct and zygoma. Its duct empties directly into the parotid duct through one tributary. The parotid duct, also known as Stensen’s duct emerges from the anterior border of the gland, travels anteriorly parallel to the zygoma, until it turns abruptly towards the buccinator muscle, piercing it and entering the oral cavity opposite the second upper molar tooth.


The SMG is the second largest major salivary gland, weighing 7–16 g and located in the submandibular triangle, bounded superiorly by the inferior edge of the mandible and inferiorly by the anterior and posterior bellies of the digastric muscle ( Fig. 37.1 ). The SMG has both mucous and serous cells that empty into ductules, which in turn empty into the submandibular duct. The submandibular ducts or Wharton’s ducts emerge anteriorly and course deep to the lingual nerve and medial to the SLG until each duct opens lateral to the lingual frenulum in the floor of the mouth behind the lower incisor tooth. SMGs comprise a mixture of mucous and serous components. Although the serous acini predominate, the proportion of both components may vary among lobes. SMG saliva is viscous and rich in glycoproteins, sulfated cystatins and neuronal and epidermal growth factors.
The smallest of the major salivary glands is the SLG, weighing 2–4 g and consisting of mucous acinar cells. The SLG lies flat within the anterior floor of the mouth, superior to the mylohyoid muscle ( Fig. 37.1 ). Unlike the other major glands, the SLG lacks a true fascial capsule, which is instead covered by oral mucosa on its superior aspect. Several ducts (of Rivinus) from the superior portion of the SLG either secrete directly into the floor of mouth, via the sublingual caruncles, or empty into Bartholin’s duct that then continues into Wharton’s duct.
37.2.2
Histology of the Major Salivary Glands
The exocrine salivary glands secrete saliva from a flask-like, blind-ended secretory structure called the salivary acinus. Salivary acini are divided into three main types: serous, mucous, and mixed that convey their isotonic secretion into a network of intralobular (intercalated and striated) and interlobular (excretory) ducts ( Fig. 37.2 ).

37.2.2.1
Acini
The PG is predominantly made up of serous acini that comprise 8–12 pyramidal-like cells. Parotid acinar cells are surrounded by a distinct basement membrane and contain abundant cytoplasmic granules (zymogen granules) ( Fig. 37.3 ).

The SMG is classified as a mixed gland that is predominantly serous with tubular acini, which display a very granular eosinophilic cytoplasm. Only approximately 10% of the acini are mucinous, with large, triangular acinar cells containing central nuclei and clear cytoplasmic mucin vacuoles ranging in size. The mucinous cells are capped by demilunes, which are crescent-shaped formations of serous cells ( Fig. 37.4 A ). The intercalated ducts of the SMG are longer than those of the PG, while the striated ducts are shorter. In rodents, the SMGs comprise serous acini solely and the male glands contain high-columnar granular convoluted tubules detected between intercalated and striated ducts ( Fig. 37.4 B).

Like the SMG, the SLG has mixed acini with observable serous demilunes within the glandular tissue ( Fig. 37.5 ). Unlike the SMG, the SLG is predominantly mucinous.

37.2.2.2
Ducts
The salivary gland’s ductal system assists in electrolyte modification and transportation of saliva. A primary hypotonic saliva is secreted first through small acinar canaliculi into the intralobular intercalated ducts. The intercalated duct is comprised of low cuboidal epithelium and is partially covered by contractile myoepithelial cells that contribute to the salivary flow. Intercalated ductal cells exhibit central nuclei, small secretory granules in the apical domain of the cytoplasm and luminal microvilli that increase the exchange surface of the cell. The cytosolic granules of the intercalated ducts contain salivary components such as lysozyme and lactoferrin. The importance of the intercalated ducts lies within their homeostatic and replenishment features, where it has been widely considered to harbor salivary gland pluripotent/stem cells that give rise to acinar, myoepithelial, and ductal cells. Striated ducts are lined by a tall columnar epithelium with central nuclei and have distinguishing basal striations ( Fig. 37.6 ) due to membrane invaginations that are loaded with mitochondria. The abundant presence of mitochondria reflects their excessive energy-powered engagement in regulating the bidirectional transport and reabsorption of electrolytes between the lumen and the extracellular space. The next segment of the duct system comprises the interlobular excretory ducts that reside within the connective tissue of the glandular septae. They are responsible for continuing the reabsorption of sodium and potassium secretion, and subsequently conducting the final saliva to the oral cavity. Excretory ducts are formed by pseudostratified epithelium, which transforms into stratified structures when approaching the oral mucosa. Goblet cells are sparsely seen interspersed among the lining epithelium.

Myoepithelial cells are associated with the acinar and intercalated ducts. Via their contractile ability, myoepithelial cells play an essential role in acinar salivary secretion. Immunohistochemistry identified the dual epithelial and contractile properties of these cells by showing their ample expression of keratins and contractile proteins such as actin, caldesmon, calponin, and smooth muscle actin. Anatomically, myoepithelial cells embrace the secretory units between the acinar basement membrane and mesenchyme. In addition to their contribution to the secretory process, myoepithelial cells have also been associated with production of the basement membrane and propagation of neural stimuli ( Fig. 37.7 ).

37.2.3
Minor Salivary Glands
Minor salivary glands ranging from 1 to 5 mm in size, line the oral cavity and oropharynx. Most commonly found in the lips, tongue, buccal mucosa, and palate, each gland consists of small clusters of secretory cells with a simpler duct system, where the intercalated ducts are longer and the striated ducts are either less developed or not present and the short excretory duct delivers saliva directly into the oral cavity. Minor salivary glands can be serous (von Ebner’s), mucous (Weber’s, lateral tongue border), mucoserous anterior ventral tongue (Blandin-Nuhn). In contrast to the major glands, the minor salivary glands have little or no sympathetic innervations, and their main nervous drive is parasympathetic with cholinergic transmission ( Fig. 37.8 ).

37.3
Control of Salivary Secretion
37.3.1
Reflex Secretion of Saliva
The reflex leading to salivary secretion is initiated by chemo- and mechanoreceptors associated with oral structures; taste buds containing receptors for sour, sweet, bitter, and umami are located predominantly in the epithelium on the dorsum of the tongue while mechanoreceptors are associated with mucosal surfaces and the periodontal ligaments. Temperature and pungent substances such as capsaicin and hydroxyl- alpha-sanshool and cooling agents such as menthol can elicit reflex salivation via activation of TRP (transient receptor potential) channels expressed on trigeminal nerve endings, taste receptors, and oral keratinocytes . Afferent nerve fibers of the facial (CN VII), glossopharyngeal (CN IX), and trigeminal (CN V) nerves innervate receptors and conduct signals to nuclei in the brainstem. The nucleus of the solitary tract is innervated by the CNVII and CNIX and is connected by interneurons to the superior and inferior salivary nuclei in the medulla oblongata. The sympathetic salivary centers are located in the upper thoracic segments of the spinal cord, presumably connected to the nucleus of the solitary tract by descending neurons.
Autonomic regulation of salivary secretion has been reviewed previously. Efferent preganglionic parasympathetic nerve fibers in the chorda lingual nerve from the salivary nuclei in the medulla conduct signals to the submandibular ganglion and then postganglionic nerves innervate the SMG and SLG. The PG is supplied by efferent fibers in the glossopharyngeal (tympanic branch) nerve via the otic ganglion ( Fig. 37.9 ). Minor salivary glands are supplied by parasympathetic nerve fibers in the buccal branch of the mandibular nerve, the lingual nerve, and the palatine nerve.

Nerves from the frontal cortical areas of the brain modify autonomic nerve signaling from the salivary nuclei. This central neural activity appears to contribute towards the resting rate of salivary secretion into the mouth since salivary flow rates are lower during sleep and virtually absent during anesthesia. Both excitatory (gamma aminobutyric acid containing) and inhibitory (glycine containing) synapse with the salivary centers. Suppression of impulse traffic through the salivary nuclei by higher (limbic and cortical) centers in the brain acting through inhibitory (glycine containing) nerves can lead to reduced salivation and dry mouth as experienced during fear and anxiety. The thought or sight of food without the taste or smell of food does not appear to give rise to salivary secretion and the experience of mouth-watering may be due to contractile activity in facial muscles near the excretory ducts of major salivary glands. Salivary secretion is also reduced during sleep and this may be due to reduced impulse traffic through cholinergic neurons arising from central nuclei (pedunculopontine and lateral dorsal tegmental) associated with maintenance of wakefulness. Reduced acetylcholine release from these neurons would reduce activation of muscarinic receptor positive neurones in the salivary nuclei. Circadian alterations in such impulse traffic may play a part in the circadian pattern of resting or unstimulated salivation observed in man .
Pharmacological studies indicate that central cholinergic and adrenergic nerves appear to play a role in salivation. It appears that adrenergic agonists such as amphetamine exert an inhibitory effect on the flow of saliva through release of noradrenaline from nerves in the medulla causing activation of inhibitory α 2 -adrenoceptors rather than through a peripheral vasoconstrictive effect.
Salivary gland cells are intimately associated with the autonomic nervous system and parasympathetic and sympathetic and nerves run together with Schwann cells or as unmyelinated axons to supply acinar, ductal, myoepithelial, and smooth muscle cells in blood vessels of salivary glands. The extent of sympathetic innervation in the PG and SMG of rat, mouse, and man is greater than that present in mucous secreting glands such as the rat and human SLGs and the human minor salivary glands and in the latter appears to be directed to the vasculature rather than the parenchyma. In addition to acetylcholine and noradrenaline there are neuropeptides within autonomic and sensory nerves in salivary glands.
Studies in animal models under anesthesia have demonstrated the effects of separate sympathetic and parasympathetic nerve stimulation which respectively give rise to protein and fluid secretion. Dual nerve stimulation experiments mimic reflex stimulation of salivary secretion and the secreted saliva has an augmented protein content and large volume. Mucin secretion from mucous glands such as the rat SLG and human minor glands is dependent on parasympathetic stimulation and peptidergic stimulation with little or no involvement from sympathetic stimulation.
The effects of autonomic denervation : Researchers in the 19th century reported that salivary gland atrophy resulted from autonomic denervation. Following Interruption of the parasympathetic innervation to salivary glands secretion of saliva ceases while sympathectomy greatly reduces protein secretion. Extended periods of parasympathectomy lead to salivary gland atrophy. Since similar atrophy is not seen in knockout mice lacking both the AchM1R and AchM3R nor in atropine treated mice, there appears to be a role for other, parasympathetic noncholinergic neurotransmitters such as substance P and VIP in preventing salivary gland atrophy following denervation. Autologous transplantation of SMGs in human subjects provided an opportunity to study the effects of complete denervation. The time course of effects of denervation and reinnervation on secretion from the transplanted glands could be rationalized by comparing to earlier studies performed on animal models. After transplantation in humans, phases of temporal change in gland flow were observed. Firstly, secretion started almost immediately postoperatively and lasted for approximately 1 week and this pattern of secretion was attributed to release of neurotransmitters from degenerating postganglionic axons at neuro-effector terminals. Subsequently cessation of flow occurred for several months and this coincided with the reduction in transmitter release seen in animal studies that leads to atrophy salivary gland secretory structures. Although atrophic, the salivary acinar cells develop a hypersensitivity to blood borne catecholamines and the small amounts of acetylcholine spontaneously leaking from remaining postganglionic parasympathetic nerve terminals. The hypersensitivity led to an epiphora, which required surgical reduction of glandular tissue, the histology of which demonstrated surviving parasympathetic ganglion cells some of which were reinnervated by sympathetic nerves, which presumably had sprouted from sites on the arteries of tissue surrounding the transplant. Previous studies of animal models have demonstrated that heterologous synaptic contacts can occur by new adrenergic axons sprouting down existing parasympathetic trunks and in time developing functional connections with parasympathetic ganglionic cells. Thus, it would appear that ganglia release chemotactic signals that lead to reinnervation.
37.3.2
The Coupling of Autonomic Nerve Stimulation to Secretion in Salivary Glands
Calcium signaling . Stimulation of acinar cell secretion of saliva is primarily initiated by the neurotransmitter acetylcholine action on the basolateral muscarinic receptors Gq/11α protein coupled M3R and to a lesser extent M1 muscarinic receptors (mAchRs). This results in activation of phospholipase Cβ (PLCβ), which cleaves phosphatidylinositol 1,4, bisphosphate (PIP2) to produce diacylglycerol (DAG) and the soluble signaling molecule inositol 1,4,5, trisphosphate (IP3). IP3, then activates the endoplasmic reticulum (ER)-Ca 2 + release channels IP3 receptors (IP3Rs), which induce Ca 2 + release from the ER stores. IP3-mediated Ca 2 + release via IP3R, and the resultant depletion of endoplasmic reticulum calcium [Ca 2 + ] ER , is detected by the ER-binding protein sensor STIM1, which triggers further influx of extracellular calcium across the membrane bound, referred to as store-operated calcium entry (SOCE) of acinar cells and ensures sustained salivary secretion. Formation of the complexes of STIM1, Orai1 and TRPC1 appear to occur in regions of the plasma membrane termed caveolae, which are cholesterol-enriched microdomains, associated with receptors and ion channels.
The increase in cytoplasmic calcium originates in the apical region of acinar cells, where IP3R’s are concentrated and is propagated to other parts of the cell through calcium induced calcium release (CICR) signal amplification of the Ca 2 + signals. Ryanodine receptor 2 (RyR2) is another main intracellular Ca 2 + release channel, abundantly localized in the basal region of acinar cells. Positioned as such, it is presumed to play a role in globalization of the Ca 2 + signal in acinar cells following stimulation, by spreading Ca 2 + to sites of the ER where IP3Rs are sparse or those at a distance from the site of IP3 generation. DAG activates salivary protein secretion by triggering exocytosis at the apical plasma membrane of acinar cells through protein kinase C signaling. Noradrenaline can also trigger salivary fluid secretion through its action on α1-adrenoceptors (ADRs) which also signal through PLCβ, IP3, and Ca 2 + .
Nonadrenergic, noncholinergic signaling : Salivary acinar cells also express the purinergic receptors, G-protein-coupled P2Y and the ligand-gated, nonselective cation channel that mediates extracellular calcium influx P2X7 receptor and both can give rise to fluid secretion in an experimental setting. The majority of sustained salivary protein secretion is induced by beta adrenergic receptor (ß-AR) activation and intracellular coupling through Gs-protein-coupled adenylate cyclase activity, cAMP and protein kinase A leading to protein storage granule exocytosis. Signaling from parasympathetic nerves can also give rise to substantial salivary protein secretion via release of vasointestinal peptide (VIP) which also acts through increases in intracellular cAMP. It may be that neuropeptides modulate fluid secretory responses of salivary glands since they are released from parasympathetic nerves with acetylcholine under physiological conditions. Protein secretion in response to VIP can also be mediated by cGMP in rat and mouse salivary acinar cells which appears to act downstream of nitric oxide production by a calcium-dependent NOS of nonneuronal origin. In this signaling pathway, calcium is released from ryanodine-sensitive intracellular stores via production of the calcium mobilizing nucleotide cADP-ribose.
37.4
Cellular Mechanisms of Salivary Fluid Secretion
Salivary gland fluid secretion in response to parasympathetic stimulation occurs in two phases; acinar secretion of NaCl-rich isotonic fluid; which has the same Na + , Cl − , K + , and HCO 3 – concentrations as plasma, followed by duct modification phase, which results in the final hypotonic saliva secreted into the mouth. Fluid secretion is initiated by the action of the parasympathetic postganglionic transmitter acetylcholine on the acinar M3 muscarinic receptors (M3R) and involves intracytoplasmic calcium [Ca 2 + ] i increase, that: coordinates the action of key channels and transporters, mediate Cl − secretion, and drive water movement ( Fig. 37.10 ).

In resting acinar cells, the basolateral Na + /K + -ATPase exploits the energy of ATP hydrolysis to mediate an inward 2K + and outward 3Na + transport against its electrochemical gradient. Beside generating the high extracellular low intracellular Na + concentrations and low extracellular high intracellular K + concentrations necessary to maintain the resting membrane potential of all cells, the Na + gradient will drive chloride ion (Cl − ) entry and accumulation, four- to fivefold above its electrochemical equilibrium. At the expense of the great Na + concentration gradient, the basolateral Na + K + 2Cl − cotransporter (NKCC1) undergoes three cotransport cycles, allowing the coupled entry of 3Na + , 3K + , and 6Cl − ions into the acinar cell from the interstitium.
Importantly, the use of NKCC inhibitors demonstrated that NKCC activity mediates about 70% of the Cl − uptake that is secreted across the luminal membrane to drive secretory glands fluid secretion. The central role of NKCC1 in salivary gland fluid and electrolyte secretion was further established by deletion of the Nkcc1 gene in mice, which resulted in about 70% inhibition of salivary secretion. As for the other two cations accumulated by NKCC1, Na + is transported to the outside of the cells via Na + , K + -ATPase and K + , via K + channels. Two basolateral K + channels, have been identified, a Ca 2 + -activated K + channel known as IK1 or SK4 and a Ca 2 + – and voltage-activated channel named maxi K or Slo. Knockout (KO) of either of them does not affect saliva secretion, whereas double KO of these two K + channels significantly reduces it, suggesting that the accumulated K + ions are transported to the outside of cells via both channels.
The NKCC1 inhibition experiments revealed that the residual 30% of fluid secretion essentially depend on a surrogate mechanism of Cl − accumulation. Indeed, Cl − uptake can be alternatively dependent on the coordinated activities of Na + /H + and Cl − /HCO 3 – basolateral exchangers. The cytosolic reaction between H 2 O and CO 2 , catalyzed by carbonic anhydrase (CAII) will yield H + and HCO 3 – . The newly produced HCO 3 – will be exchanged to Cl − by the Cl − /HCO 3 – exchanger (AE2) and cells remove excess H + by the Na + /H + exchanger (NHE1).
Following stimulation and increased [Ca 2 + ] i salivary secretion starts with activation of the apical Cl − channel transmembrane member 16A (TMEM16A). TMEM16A activation will release Cl − into the central acinar lumen, which will be followed by sodium movement by a paracellular course through the tight junctions. It is important to note that the muscarinic Ca 2 + -dependent fluid secretion was completely abolished in the salivary glands of TMEM16A null (Tmem16A −/−) mice, concluding the essential role played by this channel in fluid secretion in the adult salivary glands. Typically, the transcellular accumulation of NaCl ions in the acinar lumen will result in the obligatory osmotic water flow and cell shrinkage. As a result of rapid shrinkage of the acinar cells, the paracellular space between acinar cells as well as the permeability of the intercellular junctions increase, which permits sustained secretion of saliva via the paracellular pathway. Agonist-induced cell shrinkage is momentary and followed by volume increase, which rely partly on the intracellular free Ca 2 + -levels and uptake of osmotically active particles such as K + , Na + , and Cl − via NKCC1, NHE1, and AE2.
Although water can cross the membrane bilayer, water flow in secretory cells is facilitated by the water channels; aquaporins (AQPs). The luminal membrane of acinar cells express AQP5, which plays an indispensable role in exocrine glands’ fluid secretion by providing highly permeable water channels. This was confirmed by the: (i) 60% reduction in secretion observed in the AQP5 KO mice, (ii) aberrant trafficking of AQP5 in Sjögren’s syndrome patients, (iii) markedly decreased labelling intensity of AQP5 in severely damaged irradiated SGs, and (iv) disrupted trafficking of the water channel in Streptozotocin-induced diabetic mice, featuring xerostomia. In addition, since AQP5 appears to regulate the water permeability of the paracellular pathway, its deletion disrupted integrity of the tight junction and reduced the paracellular water permeability.
After acinar fluid secretion, saliva enters the duct modification phase, whereby salivary ducts secrete K + and HCO 3 – and reabsorb Na + and Cl − . Similar to acini, the Na + /K + -ATPase generate the energy needed for moving electrolytes across the ductal cells into the basolateral interstitium. Na + will be reabsorbed from the luminal saliva via the apical Na + channel ENaC. Another two Na + /H + apical exchangers play a similar but less significant role in Na + withdrawal, the NHE2 and the NHE3 antiporters. Cl − reabsorption will take place via the apical Cl − /HCO 3 – exchanger and a cyclic adenosine monophosphate (cAMP)-dependent Cl − channel also known as CFTR. Large amounts of sodium and chloride will then be transported out of the ductal cell and into the glandular interstitium via the basolateral Na + /K + -ATPase and Cl − channels, respectively.
The source of K + in the final modified saliva is believed to be the apical K + /H + exchangers and K + -HCO 3 – cotransporters in the intralobular and interlobular ducts. Moreover, the HCO 3 – accumulated in the cytosol via the basolateral Na + /HCO 3 – exchanger (NBCeB) will enter the lumen in exchange for Cl − at the apical Cl − /HCO 3 – exchanger (slc26a6).
37.5
Vesicular Secretion of Proteins by Salivary Cells
Acinar cells secrete most of the protein detected in saliva including α-amylase, mucins proline-rich proteins, histatins, cystatins, immunoglobulin A (IgA), and many other proteins with a variety of properties. Salivary model systems established from cell lines, parotid tissue slices and freshly isolated parotid cells have been used to investigate the multiple pathways of protein secretion in acinar cells. Four main modes of vesicular protein secretion have been identified: regulated, constitutive, constitutive-like, and transcytosis to the apical membrane of acinar cells.
The regulated secretion pathway is the classical pathway of exocrine cells, accounting for 80%–90% of total protein secretion. In this pathway, fusion of secretory granules with the apical plasma membranes and protein secretion are regulated in response to extracellular stimuli. Stimulation of beta-1 adrenoceptors causes a morphologically obvious depletion of storage granules from acinar cells in most salivary glands studied and this was visualized in live cell preparations by Segawa et al. and in green fluorescent protein labeled live mice by Weigert. The fusion of storage granules with the apical membrane of acinar cells is dependent on the interaction of apical membrane SNARE proteins and secretory granule VAMP proteins. More recent studies have visualized the role of actin filaments in exocytosis. The packaging of proteins into storage granules at high concentrations requires accumulation of charge shielding calcium and higher concentrations are required to allow packaging of salivary mucins which are highly sialylated and sulfated. Mucins are large highly glycosylated proteins containing many posttranslational modifications including sulfation and sialic acid that impart numerous negative charges. On exocytosis mucin unfolds and becomes hydrated via the loss of calcium from the core structure, a process requiring bicarbonate ion interaction with calcium.
Constitutive secretion has been described from studies of parotid acinar cells and appears to originate in the trans-Golgi network (TGN) rather than in the secretory granules. Secretion of small amounts of salivary proteins via constitutive secretion occurs continuously and in absence of stimulation. The apical translocation of the AQP5 water channel independently of stimulated salivary protein secretion may represent an example of the constitutive secretory pathway in parotid acinar cells. In the constitutive-like secretion pathway , immature granules bud from the TGN and undergo direct exocytosis in response to secretagogue stimulation as do mature granules in the regulated secretory pathway autonomimetics. Under less stimulated conditions, immature granules mature and condense their core over time. Although salivary proteins secreted into the oral cavity via the regulated or constitutive-like pathways undergo apical secretion, basolateral secretion can take place and involves a separate vesicle pathway that originates in the TGN. Vesicular transcytosis involving the epithelial polymeric immunoglobulin receptor (pIgR) delivers Immunogobulin A across salivary cells and into saliva as SIgA, a complex of dimeric IgA, J chain, and Secretory Component, the cleaved product of pIgR. Both parasympathetic and sympathetic nerve-mediated stimuli upregulate secretion of IgA into saliva (see Ref. ).
Biogenesis of secretory granules is initiated in the TGN, then undergo a poorly defined process of maturation during which salivary protein contents are condensed, for example, amylase, parotid secretory protein (PSP) and proline-rich proteins and nongranule proteins and excess membrane are removed. To reach the apical plasma membrane, SGs must traverse a terminal web of actin filaments involving myosin motor proteins and actin rearrangements, until they undergo exocytosis over the next 24 h in response to a meal.
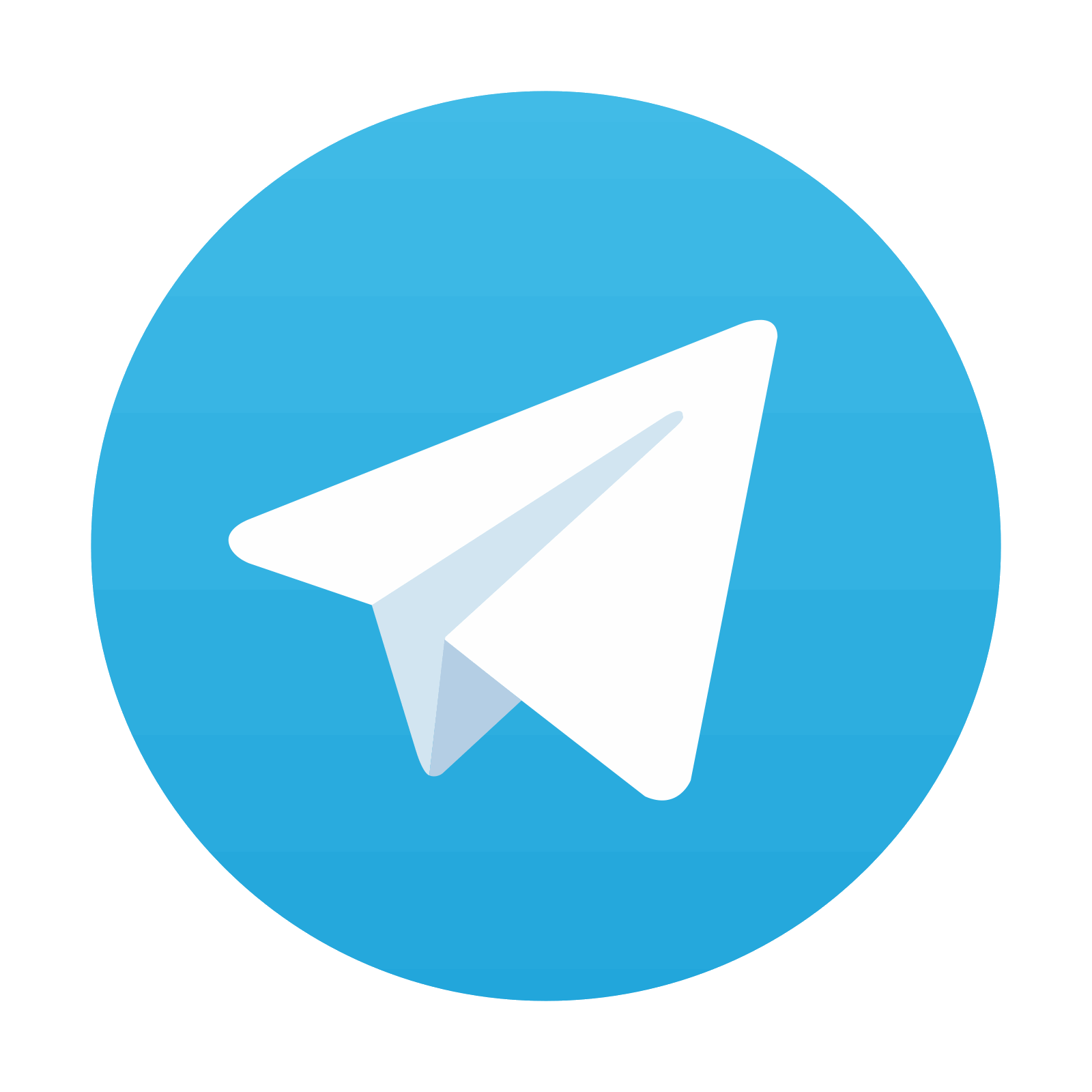
Stay updated, free articles. Join our Telegram channel
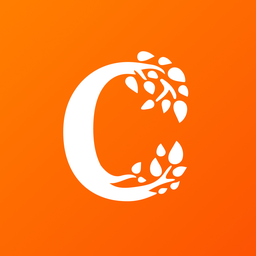
Full access? Get Clinical Tree
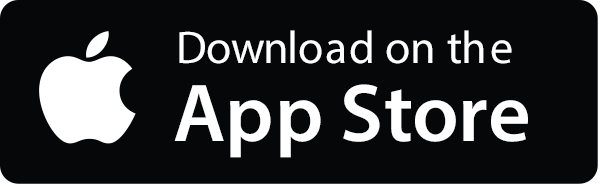
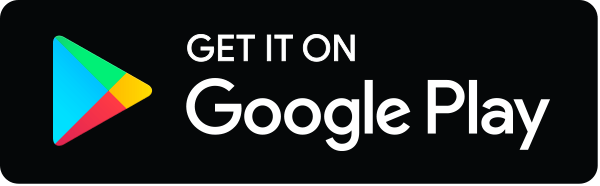