Modality
Cost
Radiation
Nephrotoxicity
Other side effects
Imaging medium
US
+
–
None
None
Sound waves
CT
++
+++
(generally performed as a three-phase study)
+ (eGFR >/= 40–45 poses minimal risk)
None
Ionizing radiation
MRI
+++
–
None
Interaction with implanted devices and ferromagnetic objects,
NSF in ESRD
Radiofrequency waves in a strong magnetic field
PET/CT
++++
++
None
None
Ionizing radiation (CT) and gamma rays emitted from radiotracer
IR
++++
+++
+
Bleeding, organ injury, infection (depending on the procedure)
Ionizing radiation and/or sound waves
Imaging Modalities
Ultrasound
US generates diagnostic images by sending sound waves from a probe placed on the region of interest (ROI) (using acoustic gel to enhance sound wave transmission) and receiving the reverberated sound waves using the same probe device. The US images are updated in real time for review by the operator who chooses selected images for capture and documentation of key structures. Sound waves pass through fluid and solid organs, yielding diagnostic images of these structures, but fail to transmit through gas and skeletal structures and fail to render these structures sonographically. Because sound waves emanate only centimeters into the body from an operator-directed probe, US is a focused exam where the US technologist targets the ROI according to the provided history. The procedure is relatively labor and time intensive and operator dependent compared with other imaging modalities, and procedures are generally best tailored to the clinical question. For example, in the setting of right upper quadrant pain, a “right upper quadrant” US is typically ordered, which directs the technologist/operator to focus on the liver, biliary system, and gallbladder. In the setting of pre- and post-LT patients, imaging the hepatic vasculature is also important and this requires ordering “Doppler ultrasound” (DUS).
DUS capitalizes on the Doppler effect, which represents the change in frequency of a (sound) wave for an observer (a vessel) moving relative to its source (the transducer). Color Doppler US uses this principle to depict blood flow in color-encoding terms with increasingly intense red and blue colors reflecting flow toward and away from the transducer, respectively and conventionally. Additionally, spectral Doppler generates a velocity-versus-time graph depicting blood flow at a point defined by the operator. As such, DUS offers the ability to evaluate the hepatic vasculature in the pre- and post-LT settings to evaluate (1) the evidence of portal hypertension; (2) portal, superior mesenteric, splenic, and hepatic venous patency; (3) hepatic arterial stenosis or thrombosis; and (4) flow derangements in the setting of transplant rejection and other post-LT complications.
Computed Tomography (CT)
CT generates images by jointly rotating an X-ray tube and X-ray detector around a circular gantry. The table moves the patient through the gantry while the tube and detector spin and the CT computer system deconvolutes the accumulated data into diagnostic images through an image reconstruction process. Filtered back projection (FBP) was the original image reconstruction methodology, while most modern scanners rely on iterative reconstruction to convert source data into diagnostic CT images incurring a fraction of the radiation dose required by FBP. In addition to the reconstruction algorithm, the CT radiation dose depends on the number of series obtained. For example, liver imaging for HCC or transplant surveillance generally requires “triphasic technique,” which means obtaining precontrast, arterial phase, and portal phase sets of images, as well as delayed postcontrast images. The risk-to-benefit ratio in the LT setting generally argues in favor of proceeding with CT scanning, given the very small risk of ionizing radiation complications and the great diagnostic yield.
Nephrotoxicity from iodinated contrast is another consideration, which is generally considered in the context of the estimated glomerular filtration rate (eGFR), which is calculated from serum creatinine and several other patient parameters. Contrast-induced nephrotoxicity (CIN) has experienced a long history of controversy, and current work has significantly curtailed the risk. With a stable GFR of at least 45 mL/min/1.73 m2, the CIN risk is essentially nonexistent; with GFR between 30 and 44 mL/min/1.73 m2, the CIN risk is minimal (odds ratio = 1.40); and when the GFR is below 30, the CIN risk is substantial (odds ratio = 2.96) (Davenport et al. 2015). Clearly, patients already committed to a long-term dialysis have nothing to risk from iodinated contrast (as long as they are not allergic). The only other contraindication to iodinated contrast is acute renal failure, although the nephrotoxicity risk is low (ACR 2013) .
Magnetic Resonance Imaging (MRI)
MRI generates diagnostic images by exploiting the behavior of protons subjected to radiofrequency energy in a strong magnetic field. Hydrogen protons in the body are magnetized by the strong magnetic field of the MR system and shift between high- and low-energy states as radiofrequency energy is applied to the system. These energy shifts release energy that ultimately provides the information used to generate MR images. The basic components include the MRI system, which is usually a superconducting magnet in a solenoid configuration housed in a cylindrical gantry; the gradient system, which modifies the magnetic field to provide spatial localizing information; and the radiofrequency coils, which send and receive the radiofrequency energy to interact with the protons and receive their emitted energy, respectively.
While MRI is generally considered a safe imaging modality and avoids the potentially harmful effects of ionizing radiation, a few risks need to be heeded. The strong static magnetic field interacts with ferromagnetic objects, potentially accelerating them toward the center of the magnetic field. Safety provisions – in the form of access restriction, education, metal detectors, etc. – are undertaken to avoid the projectile effect, whereby an extrinsic ferromagnetic object (i.e., oxygen tank) experiences the projectile effect. Careful patient screening protects patients from dislodgement of implanted devices (i.e., cochlear implant, aneurysm clip, etc.) and device malfunction (i.e., cardiac pacing device). Weaker time-varying magnetic fields, used to encode spatial information, can induce electrical currents in conducting devices and may cause neuromuscular stimulation. The thermogenic effect of the radiofrequency energy converts some of the applied energy to heat, and heating sensations are occasionally experienced. Radiofrequency energy also induces electrical current in wires and leads, potentially triggering arrhythmias (Dill 2008). Practically speaking, the vast majority of implanted devices are MRI compatible but require confirmation of the manufacturing information. Foreign bodies almost never contraindicate MRI, except when lodged in or in close proximity to either the central nervous system or the orbit.
Positron Emission Tomography (PET/CT)
PET/CT houses both a positron emission tomography and a computed tomography scanner with a single-gantry system. Consequently, images from both systems are sequentially obtained, correcting for gamma-ray attenuation differences throughout the body and facilitating fusion and cross-correlation of functional information (from PET) and anatomic information (from CT). PET scanning involves localizing the gamma rays produced when an injected radionuclide – usually fluorodeoxyglucose (FDG) – emits a positron colliding with and annihilating an electron-positron pair. The FDG serves as a glucose analog, localizing in highest concentrations in areas of the greatest metabolism – generally tumors and inflammation. Normoglycemia is important to preempt competitive uptake between FDG and endogenous glucose and maximize FDG metabolism. Ionizing radiation constitutes the chief risk associated with PET/CT. While the radiation dose contribution from the CT component of the examination is generally relatively low, the additive radiation dose from CT and PET is not insignificant. PET/CT is not included in the routine evaluation of hepatobiliary malignancies, as outlined in the National Comprehensive Cancer Network Guidelines Version 2.2015. However, PET/CT potentially plays a role in assessing metastatic disease.
Interventional Radiology (IR)
Interventional radiology (IR) procedures are generally undertaken to address a complication and occasionally for diagnostic purposes. IR procedures encompass a wide spectrum from image-guided biopsy and drainage to percutaneous biliary procedures to vascular interventions, such as catheter-directed angiography and chemoembolization and transjugular intrahepatic portosystemic shunt (TIPS) placement. Image-guided biopsy and drainage procedures are usually performed with either CT or US guidance, depending on lesion and surrounding structure visibility or conspicuity. Biliary and vascular interventional procedures are generally performed in an interventional suite guided primarily with fluoroscopic visualization supplemented by ultrasound. In addition to the risks of ionizing radiation – which can be substantial, depending on the duration of the procedure – risks related to procedural invasiveness and the structures involved must be considered. Potential procedural complications include bleeding, organ injury, infection, biliary leak, bowel perforation, and pneumothorax.
Pretransplantation Imaging
Chronic Liver Disease and Malignancy
Candidates for LT with chronic liver disease require periodic imaging surveillance for HCC, which is typically performed with US, although CT or MRI are used in some North American transplant centers routinely or when US images are prohibitively limited (i.e., body habitus, limited positioning and breath holding, etc.). For definitive diagnosis or in problem-solving situations, MRI is usually the favored imaging study, although multiphasic CT is a reasonable alternative. With MRI contraindications, CT (with and without intravenous contrast) serves as a reasonable alternative. US images are obtained systematically with representative transverse and longitudinal images recording anatomic landmarks to ensure that all relevant anatomy is covered (see Fig. 1).


Fig. 1
Representative US images. (a) Transverse gray-scale US image near the level of the hepatic vein confluence (arrow) shows the normal homogeneous appearance of the liver. (b) Longitudinal gray-scale image through the right hepatic lobe and gallbladder (arrow) shows the normal appearance of a portal vein branch with echogenic walls (open arrow). (c) Corresponding longitudinal color DUS image through the same portal venous branch demonstrates hepatopetal flow with red signaling flow toward the US probe
The normal liver appears homogeneously hypoechoic (or dark) traversed by anechoic (even darker) tubular biliary and vascular structures. Portal venous branches discriminate themselves from other fluid-filled structures by their echogenic (bright) walls. With the progression of chronic liver disease and inflammation, a number of sonographic changes occur. Heterogeneity and coarsening of the hepatic echotexture with increasing echogenicity and potentially hepatomegaly (over 15.5 cm in the midclavicular line) develop early but are generally insensitive and not reliable (Tchelepi et al. 2002). With ensuing parenchymal destruction, fibrosis, and regeneration, surface nodularity becomes apparent and signals underlying cirrhosis (see Fig. 2).


Fig. 2
Sonographic features of chronic liver disease and cirrhosis. (a) Longitudinal gray-scale US image through the left hepatic lobe shows coarsening and heterogeneity of the liver parenchyma in a patient with chronic viral hepatitis. (b) Transverse gray-scale US image reveals the same findings
Supporting findings of portal hypertension include ascites, which appears as anechoic fluid pockets, splenomegaly, portosystemic collateral vessels, and enlargement of the main portal vein (over 13 mm) (see Fig. 3) (McGahan and Goldberg 2008).


Fig. 3
Sonographic features of portal hypertension. (a) Gray-scale US image shows nodularity of the liver surface indicating cirrhosis, which is outlined by anechoic ascites. (b) Longitudinal gray-scale image through the spleen with calipers placed yielding a measurement of 19.3 cm in a patient with portal hypertension
DUS adds additional diagnostic information in the setting of cirrhosis and portal hypertension. Diminished and ultimately reversed portal venous flow is reflected using color DUS and spectral DUS images (see Fig. 4).


Fig. 4
DUS of portal hypertension. (a) Color DUS image through the porta hepatis reveals hepatofugal portal venous flow reflected by the blue color with the spectral tracing (arrow) recording the flow velocity. (b) Longitudinal color DUS image through the left hepatic lobe showing a large paraumbilical collateral vessel directing flow toward the US probe and away from the liver
Associated hepatic arterial hemodynamic derangements are also characterized sonographically using DUS. Hepatic venous waveforms gradually transform from a triphasic pattern in the normal liver to a monophasic waveform with the progression of cirrhosis.
US is highly specific (over 90 %) but less sensitive (approximately 60 %) for HCC and is the usual primary imaging screening modality (Bennett et al. 2002; Colli et al. 2006; Singal et al. 2009). The AASLD recommends US surveillance every 6 months in patients with cirrhosis. HCC is discriminated from the background liver by its heterogeneity and difference in echogenicity compared with the background liver parenchyma (see Fig. 5).


Fig. 5
US of HCC . (a) Longitudinal and transverse gray-scale images through a heterogeneous, cirrhotic liver demonstrate a heterogeneously hyperechoic solid mass indicated by the calipers. (b) Longitudinal and transverse gray-scale images in the same patient obtained more caudally reveal a large, exophytic isoechoic mass (arrows) with overlying ascites (open arrow). (c) Transverse gray-scale image shows a heterogeneous hyper- and hypoechoic mass deforming the capsular surface in the same patient with multifocal HCC
With the liver disease progression, however, HCC conspicuity generally fades as the background liver often becomes increasingly heterogeneous and echogenic. This is exacerbated by the variable sonographic appearance of HCC, ranging from hypo- to hyperechoic (McEvoy et al. 2013). Size criteria provide some guidance with new nodular lesions exceeding 1 cm more likely corresponding to HCC. Nodular lesions below 1 cm undergo surveillance with 3-month-follow-up US for 2 years, while those exceeding 1 cm should undergo contrast-enhanced MRI or CT for definitive diagnosis (Bruix and Sherman 2005; Bruix and Sherman 2011). Associated portal and/or hepatic venous invasion is demonstrated by replacement of the anechoic lumen by relatively echogenic material with tumor thrombus exhibiting DUS evidence of vascularity.
MRI covers the entire abdomen and portions of the lower chest and upper pelvis, depending on patient size and technical parameters. Various pulse sequences are obtained exploiting the rich tissue contrast capabilities of MRI and include T1-weighted images (T1WIs) with sensitivity to microscopic fat and T1WIs with sensitivity to iron; T2-weighted images (T2WIs) with sensitivity to water bound to macromolecules (high content in solid neoplasms); T2WIs with sensitivity to free water (i.e., ascites, bile, etc.); diffusion-weighted images (DWIs) with sensitivity to hypercellular lesions; and pre- and postcontrast dynamic images with sensitivity to paramagnetic substances (i.e., hemorrhage and gadolinium), vascular structures, and solid lesions/neoplasms (see Fig. 6).


Fig. 6
Representative MRI images. (a) Axial T2WI with fat suppression renders tissues with higher contents of bound water (i.e., the spleen, kidneys, and malignant lesions) relatively hyperintense. (b) Axial T1W inphase image – the liver appears relatively hyperintense under normal circumstances. (c) The axial T1W fat-suppressed image is the precontrast component of the dynamic contrast-enhanced portion of the study, and only paramagnetic substances are hyperintense (i.e., gadolinium, hemorrhage, melanin, etc.). (d) The axial T1W fat-suppressed arterial phase image is designed to render hypervascular lesions (i.e., HCC, focal nodular hyperplasia, etc.) hyperintense
Most of these pulse sequences are performed with breath-hold technique within 20 sec or so, although some employ respiratory gating to trigger image acquisition only during the quiescent phase of respiration to eliminate breathing motion artifact.
The normal liver appears homogeneously T1-hyper- and T2-hypointense and exhibits a characteristic enhancement pattern. During the arterial phase, the liver enhances modestly in proportion to the relatively small contribution of the hepatic artery. In the subsequent portal venous phase, the liver enhances maximally. The delayed postcontrast appearance of the liver depends on the contrast agent used. Extracellular agents are cleared by the kidneys fairly rapidly and hepatic enhancement fades. However, combination agents are also metabolized by the liver, which induces progressive hyperintensity over 20 min or so. Consequently, non-hepatocellular lesions, including HCC (except for 10–20 % of well-differentiated HCCs) (Kim et al. 2009; Frericks et al. 2009), fail to take up the contrast agent and appear hypointense against the hyperintense background of the normal liver parenchyma (see Fig. 7).


Fig. 7
Appearance of non-hepatocellular lesions on hepatobiliary phase images. An axial T1W fat-suppressed image obtained 20 min after the administration of combination contrast agent isolates non-hepatocellular lesions – breast cancer metastases, in this case (arrows) – as hypointense against the normal hyperintense parenchymal background. Hyperintense contrast is excreted into the biliary system (open arrow) on delayed images
This phenomenon is a function of the transporter molecule organic anion-transporting peptides (OATP) located on the normal hepatocyte basolateral membrane. While agents with hepatic metabolism provide this diagnostic benefit, cirrhosis limits its utility because of the limited contrast agent uptake and variable OATP expression (Chanyaputhipong et al. 2011), so extracellular agents are preferred at most centers for evaluating cirrhotic livers.
MRI clearly depicts hepatic morphologic changes in chronic liver disease. Trophic changes generally constitute the earliest imaging signs of underlying liver disease and include caudate lobe and lateral segmental hypertrophy and right lobe and medial segment atrophy. These changes are reflected by enlargement of the periportal space, gallbladder fossa, and major interlobar fissure and an increased ratio of the length of the caudate lobe to the right lobe. With progressing inflammation and evolving cirrhosis, nodularity and fibrosis develop leading to a cobblestone appearance. Nodular regenerative and dysplastic tissue generally exhibits signal characteristics similar to normal liver, although some nodular lesions appear T1-hyper- and T2-hypointense. Reticulated fibrosis surrounding the nodular lesions is characteristically T2-hyperintense with progressively delayed enhancement (see Fig. 8) (Ito et al. 2002; Gupta et al. 2004).


Fig. 8
MRI appearance of chronic liver disease and cirrhosis. (a) Axial T2W MRI image shows the nodular liver contour indicating cirrhosis with trophic changes characteristic of chronic liver disease – caudate and lateral segmental hypertrophy and medial segment and right lobe atrophy. Splenomegaly and gastric varices corresponding to signal voids (arrow) and trace ascites (open arrows) indicate portal hypertension. (b) Postcontrast fat-suppressed T1W MR image shows the hepatic morphologic derangements, splenomegaly, and varices, along with a TIPS (arrow). (c) More cephalad postcontrast fat-suppressed T1W MR image at the same level as (a) shows the same gastric varices after enhancement, along with gastrohepatic varices (arrow) and the mid-segment of the TIPS (open arrow)
MRI performance in detecting HCC has varied in the literature as a function of technological innovation and lesion size considerations. With technical improvements in MR systems over the years, the MRI accuracy has clearly improved, yet research substantiates limitations in evaluating small lesions. Forner et al. (2008) reported high specificity (93.1 %) but relative insensitivity (61.7 %) for HCC lesions smaller than 2 cm. Marin et al. (2009) observed similar performance with MRI sensitivity and specificity depending on lesion size: 42.8 and 100 % when <1 cm, 83.3 and 80 % when 1–2 cm, and 100 and 91 % when >2 cm. MRI features are well established, and arterial hyperenhancement is a virtual sine qua non of HCC diagnosis and figures prominently into the Liver Imaging Reporting and Data System (LI-RADS) developed and promoted by the American College of Radiology (ACR) to “standardize the reporting and data collection of CT and MR imaging for hepatocellular carcinoma” (ACR 2014) (see Table 2).
Categorization | LR-1 | Definitely benign |
LR-2 | Probably benign | |
LR-3 | Intermediate probability | |
LR-4 | Probably HCC | |
LR-5 | Definitely HCC | |
LR-5 V | Tumor in the vein | |
LR-M | Probably malignant, not specific for HCC | |
LR treated | Treated observation | |
Applicable imaging modalities | CT with extracellular agent | |
MRI with extracellular agent | ||
MRI with combination agent | ||
Imaging features assessed | Arterial phase hyperenhancement | |
Washout | ||
Diameter | ||
Diameter increase (growth) | ||
Capsule appearance | ||
Visibility at surveillance US | ||
Ancillary features | Hepatobiliary phase hypointensity | |
Mild to moderate T2-hyperintensity | ||
Restricted diffusion | ||
Distinctive rim | ||
Corona enhancement | ||
Mosaic architecture | ||
Nodule-in-nodule appearance | ||
Intralesional fat | ||
Lesional fat sparing | ||
Blood products | ||
Diameter increase less than threshold |
Additional major features include washout (the lesion becomes hypointense compared with the liver on portal phase and delayed images), delayed-enhancing capsule, and growth. A number of ancillary features are observed – T2-hyperintensity, diffusion restriction, mosaic architecture, intralesional fat, etc. – in addition to the propensity of HCC to invade venous structures (see Fig. 9).


Fig. 9
MRI features of HCC . (a) Axial T2W MR image in a cirrhotic liver with trophic changes (lateral segmental hypertrophy and medial segmental and right lobar atrophy) reveals a mildly hyperintense lesion reflecting an increased bound water content (arrow). (b) The lesion exhibits avid heterogeneous enhancement on the arterial-phase postcontrast T1W fat-suppressed MR image. (c) The delayed postcontrast T1W fat-suppressed MR image demonstrates the typical washout pattern with the late-enhancing capsule. (d) The ADC map MR image shows lesional hypointensity reflecting decreased diffusivity due to the relative hypercellularity
While smaller lesions typically adhere to the nodular morphologic pattern, larger, more progressed lesions typically fall into three main morphologic categories: (1) solitary, (2) multifocal, and (3) infiltrative (see Fig. 10).


Fig. 10
MRI of HCC growth patterns. (a) T1W postcontrast arterial-phase image with fat suppression demonstrates two hypervascular lesions (arrows) corresponding to HCC lesions in a patient with multifocal HCC. (b) The lesions (arrows) washout on the T1W delayed postcontrast image. (c) A more caudal T1W postcontrast arterial-phase image with fat suppression reveals additional hypervascular HCCs (arrows). (d) Another T1W postcontrast arterial-phase image slightly more caudally shows additional HCCs (arrows). (e) The T1W postcontrast arterial-phase image in a different patient reveals two discrete geographic heterogeneously hypervascular regions indicting infiltrative HCC (arrows). (f) The fat-suppressed T2WI demonstrates the hyperintensity of the increased bound water content (arrows) and focal invasion of the left portal vein (open arrow)
Many of the MR features of HCC apply to CT, including arterial hyperenhancement, washout, delayed-enhancing capsule, mosaic architecture, corona enhancement, and intralesional fat (see Fig. 11).


Fig. 11
CT of HCC . (a) Axial postcontrast arterial-phase CT image reveals a large heterogeneously hypervascular lesion in hepatic segment 7(arrows). (b) A slightly more caudal postcontrast arterial-phase CT image through the lesion (arrows) shows portal venous invasion with tumor thrombus (arrowhead)
CT lacks the capability of assessing diffusion restriction and availability of contrast agents with hepatobiliary activity. CT performs nearly as well as MRI in HCC detection, but implicates ionizing radiation and its risks along with at least the specter of nephrotoxicity from iodinated contrast agents. Therefore, MRI is generally favored over CT for liver evaluation and lesion detection.
Although cholangiocarcinoma shares some of the same risk factors as HCC, its prevalence on imaging studies in the setting of chronic liver disease is far less frequent. While its imaging appearance depends on its location and growth pattern, in the setting of chronic liver disease, cholangiocarcinoma is typically intrahepatic (as opposed to the Klatskin and extrahepatic varieties) exhibiting either mass forming or infiltrative growth patterns. The sonographic appearance of the mass varies from hypo- to hyperechoic or mixed echogenicity, and upstream biliary dilatation with abrupt ductal obliteration is occasionally associated (Sainani et al. 2008). CT reveals a hypodense mass on unenhanced images that demonstrates a peripheral hypovascular and progressively centripetal enhancement pattern, reiterating its peripherally cellular and centrally desmoplastic histology (Han et al. 2002). On MR images, this often conforms to peripheral hyperintensity and central hypointensity on T2WIs with the same centripetal enhancement pattern. Because of the desmoplastic content, capsular retraction is occasionally observed. Diffusion restriction and hepatobiliary phase hypointensity are additional MRI features (see Fig. 12) (Chung et al. 2009; El Fattach et al. 2015).


Fig. 12
Imaging of cholangiocarcinoma . (a) Axial postcontrast arterial-phase CT image shows an irregularly marginated lesion (arrows) with peripheral enhancement corresponding to viable tumor cells and central hypovascularity indicating necrosis and desmoplasia. (b) T1W fat-suppressed MR image demonstrates lesional hypointensity. (c) The T1W fat-suppressed postcontrast arterial-phase MR image demonstrates the same enhancement pattern. (d) The delayed T1W fat-suppressed postcontrast MR image typifies the characteristic progressive enhancement pattern. (e) Fused PET/CT image shows marked hypermetabolism in the peripheral cellular portion of the tumor (arrows). (f) An image from a portal venogram prior to embolization isolates the lesion (arrow) due to its exclusive arterial blood supply in contradistinction to the normal liver receiving 75–80 % of its blood supply from the portal venous system
Elastography
For patients with chronic liver disease such as hepatitis B or C or fatty liver disease, standard imaging techniques are useful for identifying advanced liver disease and portal hypertension, although with low sensitivity for identifying underlying fibrosis. Diagnosing fibrosis is clinically important since hepatic fibrosis is a dynamic process potentially stopped or reversed with medical treatment. Liver biopsy, the current gold standard for diagnosing fibrosis, has many drawbacks including patient reluctance, biopsy complications, sampling error, and interobserver variability in reporting pathology results. Liver elastography, an imaging technique performed using MRI or ultrasound, quantifies fibrosis and in many cases eliminates the need for liver biopsy.
Elastography interrogates the mechanical properties of tissues based on the speed of shear wave propagation. Using MRI or ultrasound, a mechanical device deforms tissues to generate shear waves. The shear wave velocity is then measured yielding quantitative results measured in kilopascal (kPa). In general, the propagation of shear waves is faster in stiff or hard tissues. Studies have shown that an increase in liver stiffness is correlated with increasing stages of hepatic fibrosis (Yin et al. 2007; Bonekamp et al. 2009).
Various ultrasound elastography methods exist. Fibroscan®, the first ultrasound machine to perform elastography, uses mechanical push pulses to generate shear waves. While well established in the literature, Fibroscan® has drawbacks including a small tissue sample size, isolated peripheral right lobe sampling, and a high diagnostic failure rate due to patient obesity or ascites. Also, Fibroscan® is an exclusively elastography system without the capabilities of performing the standard diagnostic ultrasound study to identify liver lesions and characterize other findings.
However, many of the ultrasound vendors have added elastography to standard ultrasound machines. Rather than mechanical pulses, elastography is commonly performed using acoustic radiation force imaging to generate shear waves. The major benefit of the combined ultrasound/elastography units is the ability to combine the elastography and diagnostic ultrasound components of the study into one exam. Another advantage of this technique over Fibroscan® is a lower technical failure rate since a diagnostic ultrasound machine directs where the elastography measurement is taken optimizing measurement accuracy. Finally, ascites is generally not a limitation compared with Fibroscan®. The disadvantages include a small liver sample size, similar to Fibroscan®, and less available literature to establish fibrosis thresholds.
With MR elastography, a passive driver placed on the abdomen over the liver generates mechanical vibration to create shear waves propagating through the liver. The shear waves are measured by the equipment and generate a wave image (see Fig. 13a), which is then used to create an elastogram (see Fig. 13b, c).


Fig. 13
MR elastography. (a) Wave image that is used to generate an elastogram. A passive driver placed on the right anterior abdominal wall generates the mechanical vibration that is used to create this image. (b) Elastogram which was generated from the wave image in Fig. 1 in a patient with a normal liver stiffness of 2.2 kPa. The color map ranges from lower kPa values (blue) to higher values (red). (c) Elastogram in a patient with fatty liver with cirrhosis and stage four fibrosis. The liver stiffness values were elevated at 5.1 kPa, and the color scale demonstrates areas, which are red indicating higher liver stiffness values
Liver stiffness is measured both quantitatively in kPa and qualitatively using a color map. Four images of the liver are obtained which include a large portion of the liver to sample. The benefits of MR over ultrasound elastography include sampling much larger segments of the liver and a higher technical success rate. Ascites, obesity, and overlying bowel gas usually do not preclude performing MR elastography. Similar to the combined ultrasound/elastography units, a standard MRI exam can be performed at the same time to evaluate for liver morphology/masses and for portal hypertension. Another advantage is that liver fat and iron quantification can be performed at the same time yielding the most comprehensive noninvasive liver imaging exam available. The addition of elastography and liver fat and iron quantification to a standard MRI exam only adds about 5 min of imaging time. The disadvantages of MR elastography include possible non-diagnostic studies due to liver iron overload or motion artifact and the higher cost of MRI compared to ultrasound.
Interventional Radiology Procedures
Patients often require interventional procedures to ablate lesions threatening to exclude their suitability for LT. These procedures fall into two main categories: (1) image-guided tumor ablation and (2) image-guided transcatheter tumor therapy. The former is generally performed under either US or CT guidance utilizing radiofrequency (usually), chemical, thermal, or cryoablative techniques. The latter involves the delivery of chemotherapeutic agents, embolic particles, or radioactive materials selectively into the arterial territory supplying the tumor using fluoroscopic guidance. The technical details of these procedures are beyond the scope of this text, but the appearance of procedural complications and the post-procedural appearance of lesions deserve attention.
Image-guided tumor ablation procedures incur a number of potential complications (see Table 3).
Complications related to Rf energy and heat | Thermal effects | GI tract (potentially fatal) |
Diaphragm | ||
Biliary system (strictures and bilomas) | ||
Gallbladder (cholecystitis > perforation) | ||
Rf device and pacemaker dysfunction | ||
Postablation syndrome | ||
Complications related to electrode insertion and removal | Hemorrhage | |
Infection | ||
Tumor seeding | ||
Other complications | Vascular thrombosis and hepatic infarction | |
Pleural complications | ||
Arteriovenous fistula | ||
Skin burns |
Major complications occur with an approximate frequency of 1–2 % (Livraghi et al. 2002; Giorgio et al. 2005). Some complications demonstrate revealing imaging findings, such as bowel injury, biliary injury, bilomas and hematomas, infection, and vascular thrombosis and infarction. Bowel injury most commonly involves the colon and manifests with focal, often eccentric wall thickening with or without adjacent infiltration of the mesenteric fat, pericolonic fluid collections, and/or perforation, depending on whether perforation has ensued. Injury to the biliary tract potentially induces structuring or biloma formation. Proximal biliary dilatation is the most conspicuous sign of an underlying biliary stricture, and the underlying stricture appears as a short segment of luminal narrowing and wall thickening. US is a useful screening study, which readily identifies biliary dilatation with near 100 % sensitivity but lacks utility in stricture and mass detection (Singh et al. 2014). As such, biliary dilatation in this setting generally prompts CT and/or MRI, which has the advantage of volumetric imaging covering the entirety of the biliary tract for a panoramic overview. The CT findings are more clearly defined following intravenous contrast administration with relatively hypodense, branching tubular structures converging and tapering at the site of the stricture against the hyperdense backdrop of enhancing liver parenchyma. The strictures typically extend over a short segment (a cm or less) and feature concentric relatively mild and smooth wall thickening (as opposed to malignant strictures with longer segmental involvement and a greater wall thickness and degree of heterogeneity) (Park et al. 2004; Choi et al. 2005; Shanbhogue et al. 2011). MRI demonstrates these findings with greater tissue contrast in a number of ways and adds additional diagnostic information. In a similar fashion, dilated bile ducts appear hypointense adjacent to hyperintense, enhancing liver on postcontrast T1WIs. Additionally, T2WIs portray the biliary system as hyperintense against background tissue in a scalable fashion ranging from standard T2WIs where adjacent structure visibility provides anatomic reference points to magnetic resonance cholangiopancreatography (MRCP) where only fluid-filled structures, such as the biliary tree, are visible. Consequently, the margins and configuration of the biliary stricture are demonstrated – additional features discriminating benign from malignant strictures. Benign strictures tend to feature smooth symmetric borders with tapered margins, whereas malignant strictures typically exhibit irregular, asymmetric involvement with shouldered margins (see Fig. 14) (Soto et al. 2000).


Fig. 14
Imaging features of post-procedural biliary stricture. (a) The T-tube cholangiogram image week after liver transplantation shows focal anastomotic narrowing (arrow). (b) The MRCP image exaggerates the degree of stenosis (arrow) without the benefit of active luminal distention
The biliary excretion of a combination contrast agent affords the opportunity to positively enhance the biliary tree, providing yet another means to evaluate underlying strictures and bilomas and biliary leaks. In fact, hepatobiliary phase imaging complements the other MRI techniques in identifying and localizing bile duct injury by demonstrating abnormal contrast accumulation outside the biliary system into the hepatic parenchyma (biloma) or leaking into the peritoneal cavity (see Fig. 15) (Kentarci et al. 2013).


Fig. 15
MR imaging of biliary leak. (a) An early image from a cholescintigram shows hepatic uptake of the radiopharmaceutical and faint biliary excretion into the common bile duct (arrow) and a minimal focus of radiotracer activity in the subhepatic space (open arrow). (b) A subsequent image better demonstrates biliary excretion into the common bile duct (arrow) and increased subhepatic radionuclide accumulation (open arrow). (c) The final cholescintigraphic image shows near-complete washout from the liver with progressive radionuclide spillage into the subhepatic space (arrow) from an anastomotic leak. (d) The MRCP image shows diffuse contour irregularity throughout the peri-anastomotic bile duct (arrows) reflecting the underlying injury
Post-procedural fluid collections include bilomas, hematomas, and abscesses. Fortunately, these collections each frequently exhibit characteristic differentiating MRI features. In addition to the features described above, bilomas are typically intermediately intense on T1WIs and hyperintense on T2WIs. Also T2-hyperintense hematomas demonstrate T1-hyperintensity reflecting their methemoglobin content (Shigemura et al. 1995). Pyogenic abscesses typically demonstrate a complicated cystic imaging appearance with heterogeneously T2-hyperintense and T1-hypointense contents, complicated morphology with a multicystic or clustered cystic appearance, and reactive changes in the surrounding hepatic parenchyma. Reactive changes include edematous, T2-hyperintense signal and increased arterial enhancement (see Fig. 16) (Roth 2012).


Fig. 16
Imaging of pyogenic abscess. (a) The axial postcontrast CT image shows a wedge-shaped subcapsular hypovascular lesion (arrow) with perilesional arterial hyperenhancement. (b) Axial image from an unenhanced CT image obtained approximately 2 weeks later demonstrates interval development and superimposition of a large gas-filled collection (arrow) indicating superinfection and pyogenic abscess formation. (c) Axial image from a subsequent contrast-enhanced CT obtained a few days later after percutaneous drain placement (arrow) with decreased size of the abscess. (d) Axial contrast-enhanced CT image in a different patient shows multifocal collections (arrows) characterized by the “clustered cyst” appearance. (e) More caudal postcontrast CT image in the same patient shows a large abscess in the lateral segment. (f) Axial fat-suppressed T2WI illustrates the complexity of the abscess with heterogeneous signal intensity of the collection with debris and variable stages of tissue liquefaction
US is a good screening tool to identify potential post-procedural fluid collections and presents some specific imaging features, but often prompts MRI or CT for further characterization. Pyogenic abscesses assume a wide range of appearances from hypoechoic to hyperechoic with heterogeneity and occasional acoustic shadowing (blockage to the passage of sound waves) from intralesional gas (Power et al. 2007). Hematomas often exhibit a very characteristic lacy internal septation pattern or heterogeneous echogenicity sonographically, and bilomas tend to appear as simple unilocular, anechoic cystic lesions. Similarly, bilomas demonstrate fairly nonspecific findings on CT images and appear as well defined to slightly irregular hypodense collections. The appearance of hematomas depends on chronicity because acute hemorrhage is hyperdense – with a layering fluid-fluid hematocrit level developing over time – with density gradually diminishing with the evolution of blood products (see Fig. 17).
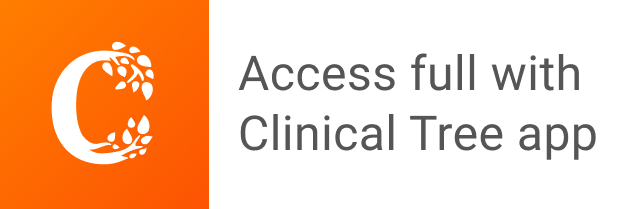