Fig. 16.1
Placement of three to four fiber-optic temperature probes (Lumasense, Santa Clara, CA) are placed at the peripheral and deep margins 5 mm from the tumor-parenchymal interface. This allows for ablation to be delivered until all probes read >60 °C. The Cool-tip® (Covidien, Valley Lab, Boulder, CO, USA) probe with hand piece is visualized in the center of the temperature probes
RFA Probe History
The placement of an uninsulated needle into tissue with application of RF energy leads to a significant buildup of electrical current at the metal/tissue interface (current density) and this leads to very high temperatures that exceed 100 °C. The resulting “burn” causes vaporization and charring within a few mm of the probe itself (much like a conventional “Bovie” electrocautery device). The initial plain electrode probe used for RFA (in cardiac ablation) was nothing more than a bare metal wire and was not able to accommodate rapid rises in impedance limiting ablation size. Modifications to the original probe include bipolar (bipolar electrodes), wet electrodes (saline perfusion), internal cooling (cooled electrodes), and enlargement of the field (multiple and expandable electrodes); allowing for increased target size [16].
Three RFA systems are currently available on the market in the USA and utilize either an electrical impedance-based or temperature-based treatment algorithm. Impedance-based systems include the Cool-tip® (Convidien, Boulder, CO, USA) and LeVeen® RF system 3000® (Boston Scientific, Natick, MA, USA). The Cool-tip® (Convidien, Boulder, CO, USA) utilizes a 480 kHz RF generator where the energy output is individually determined for up to three monopolar electrodes; the single electrode or cluster 3-electrode monopolar systems (Fig. 16.2). The system pump internally perfuses and chills each electrode to prevent charring of the target tissue; limiting the temperature increase to less than 25 °C at the tissue/probe interface but allowing for diffusion of current into the surrounding tissues and thereby creating frictional agitation and secondary heat [16, 22] (Fig. 16.3). The advantage of the 3-electrode monopolar system is less charring secondary to increased surface area [16]. The LeVeen® RF system 3000® (Boston Scientific, Natick, MA, USA), formerly Radiotherapeutics, incorporates an inverted dry umbrella design which deploys 12 tines covering a 4 cm diameter [8, 23]. This self-regulating system disperses the current over several tines and limits power output as tissue impedance rises (a Proxy for temperature) and gradually increases the power output in a technique referred to as “roll-off”.



Fig. 16.2
(a) The 1996 Circa single-tip and cluster 3-electrode monopolar straight needles, Cool-tip® (Covidien, Valley Lab, Boulder, CO, USA) probe with hand piece. The hollow probe allows for the circulation of chilled water. (b) Close up of the 1996 Circa Cool-tip® 3-electrodes in one handle with the un-insulated active tip (bracket) and centimeter markings placed at intervals along the 10–25 cm needle shaft. This probe has a 2.5 cm emitting length. (c) The updated Cool-tip™ (Covidien, Medtronic, Minneapolis, MN) single straight needle provides a 17-gauge straight trocar design for accurate placement into target tissue. (d) Close up of the Cool-tip™ (Covidien, Medtronic, Minneapolis, MN) un-insulated active tip (bracket) and centimeter markings placed at intervals along the 10–25 cm needle shaft

Fig. 16.3
The Cool-tip® (Covidien, Valley Lab, Boulder, CO, USA) generator. System internally perfuses and chills each electrode to prevent charring of the target tissue; limiting the temperature increase to less than 25 °C
The temperature-based Starburst Radiofrequency Interstitial Tissue Ablation (RITA®) system (Angiodynamics®, Queensbury, NY, NY, USA) utilizes an expandable (up to 9) array of small tines in a 16 gauge shaft providing up to 5 cm of deployment depth (Fig. 16.4). Energy is delivered by a 1500 or 1500X generator. An advantage of the RITA® system is that 5 of the 9 tines are capable of intra-operative temperature recording via a thermocouple tip [23]. As the tines expand further from the shaft there is likely to be some irregular geometric shapes and one must be cautious to avoid skip lesions when utilizing the expandable devices. The Starburst system is compatible with both wet, saline infused, and the traditional dry electrodes. Dry electrodes are limited by current density requiring longer treatment times, increased electrode surface area, multi-tine electrodes, or multiple ablations [24]. Wet electrode system, allows for current density to spread via perfused conducting saline resulting in larger ablation zone size [25]. Experimentally the use of hypertonic saline (14%) provides for a more expansive and rapidly achieved ablation zone, but this concept has never been utilized commercially [26].


Fig. 16.4
The temperature-based Starburst Radiofrequency Interstitial Tissue Ablation (RITA®) system (Angiodynamics®, Queensbury, NY, NY, USA) utilizes an expandable (up to 9) array of small tines in a 16 gauge shaft providing up to 5 cm of deployment depth
Animal studies with the hypertonic saline infused probes demonstrated reproducible and predictable zones of ablation in both acute and chronic studies [27]. All three of these systems can be used and system choice is dependent on physician preference [8]. A study conducted by Denys et al. compared the use of the systems mentioned above in the ablation of pig livers. The study found that the RITA® system provided the largest ablation volume and the Cool-tip® system produces an ovoid shape ablation zone, compared to other systems [25]. Lobik et al., evaluating cool tip and Rita in an experimental egg-white model determined that the former yielded “barrel shaped” lesions, whereas the expandable tined probes yield “Christmas-tree” shaped lesions [28]. Due to the fact that there are many different probe designs, and that “all RF probes are not created equal a consensus panel developed definitions to describe probe design and geometric lesion development [16].
In order to try to utilize MRI for probe placement and because MR signal can be altered by temperature in the target zone RFA specific MR-compatible devices were developed. The interface which develops between the MR machine and the RFA generator can be overcome by use of these specific MRI-compatible devices. Two MR compatible electrodes are available. First, the titanium Cool-tip RF system® (Covidien, Boulder, CO, USA) cools with circulating water which decreases the observed amount of charring within the tissue of the target thus improving results [2]. The second electrode is the nitinol StarBust Semi-Flex® (Angiodynamic®, Queensbury, NY, NY, USA) which provides a more flexible shaft for navigation to the target tissue in the MR limited gantry size and a larger ablation zone through the use of multiple active tines [2, 8] (Fig. 16.5).


Fig. 16.5
MR-compatible StarBust Semi-Flex® (Angiodynamic®, Queensbury, NY, NY, USA) provides a flexible shaft for navigation to the target tissue in MR limited gantry size and a larger ablation zone through the use of multiple active tines
Expansion and Development of RFA: Main Delivery Mechanisms
Laparoscopic RFA
Laparoscopic US-guided RFA is utilized in anterior tumors and tumors located within 1 cm of the bowel, as described by Sterrett et al. [3]. The laparoscopic technique permits the manipulation of organs assuring that the RFA treatment is directed only toward the intended target decreasing the risk of thermal injury to the surrounding tumor free tissue. This allows for a reduction in the number of post-procedural complications resulting from thermal injury [3, 7].
First, with the patient placed on their unaffected side, small port incisions are made to allow full abdominal insufflation. Since, US imaging lacks the ability to detect heat; we recommend the placement of peripheral temperature monitors to help aid in ablation success. If temperature monitors are not utilized end goals can be monitored by pre-set impedance or temperature goals of the RFA probes depending on the system used and by direct visualization. Gas bubbles created during the ablation cycle can interfere with the US signal thus making US an unreliable way of monitoring the treatment. Three to four fiberoptic temperature probes (LumaSense, Santa Clara, CA) are placed at the peripheral and deep margins, 5 mm from boundary between the tumor and normal renal parenchyma (Fig. 16.6). Temperature probe placement is visualized under US guidance and can be aided using 5-Fr coaxial guide needle encompassed in a radiopaque sheath (Huey, Cook Vascular, Inc., Vandergrift, PA, USA) [7, 29]. Next, the RFA probe is directed toward the target tissue under US-guidance. After all temperature probes have reached the treatment goal of 60 °C, or the impedance/temperature goal has been met the RFA probe is removed, again under US guidance [3, 29].


Fig. 16.6
Laparoscopic guided RFA is utilized in anterior tumors allowing for manipulation of vital surrounding organs. Green arrows represent fiberoptic temperature probes (LumaSense, Santa Clara, CA) placed at the peripheral and deep margins, 5 mm from boundary between the tumor and normal renal parenchyma. The white arrow represents the RFA probe directed toward the target tissue
The advantage of the laparoscopic RFA approach is the “intent to treat” described by Hui et al. and Castle et al. Percutaneous RFA can be performed by surgeons and interventionists in contrast to laparoscopic treatments which are solely performed by surgeons. The single session success rate for percutaneous approach was found to be 87% (95% CI, 82–91%) compared to 94% (95% CI, 92–96%) in the laparoscopic approach. This difference suggests that surgeons may have a more aggressive approach compared to that of interventionists [30].
Percutaneous RFA
CT-RFA
CT-RFA is best utilized for posterior tumors. The patient is first placed in either the prone or lateral decubitus position. The lateral decubitus position allows for the deflation of the dependent lung decreasing movement of the kidney secondary to respiration [31]. This decreases the likelihood of pleural space injury and pneumothorax when treating upper pole masses [2, 7]. The prone position is used most often for CT-RFA of SRM. This position increases the separation between the costal margin and iliac crest allowing for a larger insertion window. However, this also increases the length of the kidney in proximity to the lung hence limiting its use for upper pole lesions [2]. CT-RFA is best performed under general anesthesia allowing manipulation of respiratory movements during probe placement [1, 7, 19].
CT-RFA is widely available decreasing the cost of the procedure [31]. CT-RFA procedures can be done at outpatient centers lowering the cost of hospital stay and resultant complications. Compared to the laparoscopic approach CT-RFA has fewer complications since laparoscopic insertion and insufflation is avoided [7]. Disadvantages include gantry-size limited access to the patient, exposure to ionizing radiation, and lack of treatment endpoint via real-time temperature monitoring [19, 31].
MR-RFA
MR-RFA was first described by Anzai et al., in 1995 for the treatment of brain tumors [32]. In 2003, Gervais and Mayo-Smith et al. reported successful MR-RFA for renal tumors [32]. In recent literature Lewin et al. provides expertise on renal MR-RFA procedures, first introduced in 1998 [2, 7]. There are several advantages of MRI-RFA compared to CT guided treatment; most notably the lack of patient radiation exposure [2, 33]. MRI allows for increased spatial resolution and amplification of soft-tissue elements [2]. The use of MRI allows ablation of difficult-to-access lesions with trajectory limits in close vicinity to vital structures especially tumors treated near the diaphragm. These tumors are not readily accessible by CT guidance due to triangulation limits or by US due to air-artifact resulting in the increased incidence of pneumothorax [2]. More than one ablation confirmation is not possible in CT-guided RFA since contrast is needed to visualize residual tumor cells, which takes time to clear. Once contrast is injected subsequent ablation success cannot be confirmed due to the obstruction of the lingering original contrast material. As many as four ablation sessions have been reported for successful CT-RFA treatment. In contrast, MR allows for the immediate detection of post-ablation residual tumor by the presence of T2-weighted MR isointense or hyperintense signals. If residual tumor is observed, the provider can perform further ablation cycles within the same session. This eliminates the need of additional visits which would require further patient repositioning and exposure to contrast media [2, 33, 34]. It has been reported that 92–100% of MR-ablations can be achieved successfully in one session [34].
Real-time monitoring can also be achieved via MR fluoroscopy using rapid gradient echo sequences. Real-time monitoring more readily allows for the identification of a therapeutic end-point and the manipulation of the thermal ablation zone during the procedure. However, the interface of the MRI scanner presents a challenge for continuous imaging. This can be overcome by the use of intermittent MR scanning between ablations [2]. Disadvantages of MR-RFA include limited gantry size, procedure length, machine availability, equipment cost, and the need for MRI compatible sensors (as discussed earlier) [1, 31].
Cone-Beam CT
This system utilizes a large rotating C-arm and digital fluoroscopy. Manipulation of the C-arm under fluoroscopy creates a 3D model of the target allowing for precise ablation (Fig. 16.7). The trajectory is then projected onto the patient allowing for further guidance for placement of the needle along the trajectory. Once the needle is placed, further imaging is obtained via rotational angiography within seconds. This confirms needle placement and allows for further manipulation of the trajectory [1] (Fig. 16.8). A study, performed by Cheng et al., compared patient radiation exposure during cone-beam CT procedures versus conventional CT techniques in RFA ablation. Patients who underwent cone-beam ablations sustained a lower radiation dose compared to the conventional technique (P < 0.5), while having comparable treatment success [35]. This system is limited by respiratory movement since the point in the respiratory cycle where the images are obtained must be identical to that of needle placement. This can be overcome by having the patient hold end expiration or utilizing general anesthesia [1].



Fig. 16.7
Room set up f or Cone-beam CT RFA. Notice the large gantry size allowing room for needle placement. Manipulation of the large C-arm under fluoroscopy creates a 3D model (arrow) of the target allowing for precise ablation

Fig. 16.8
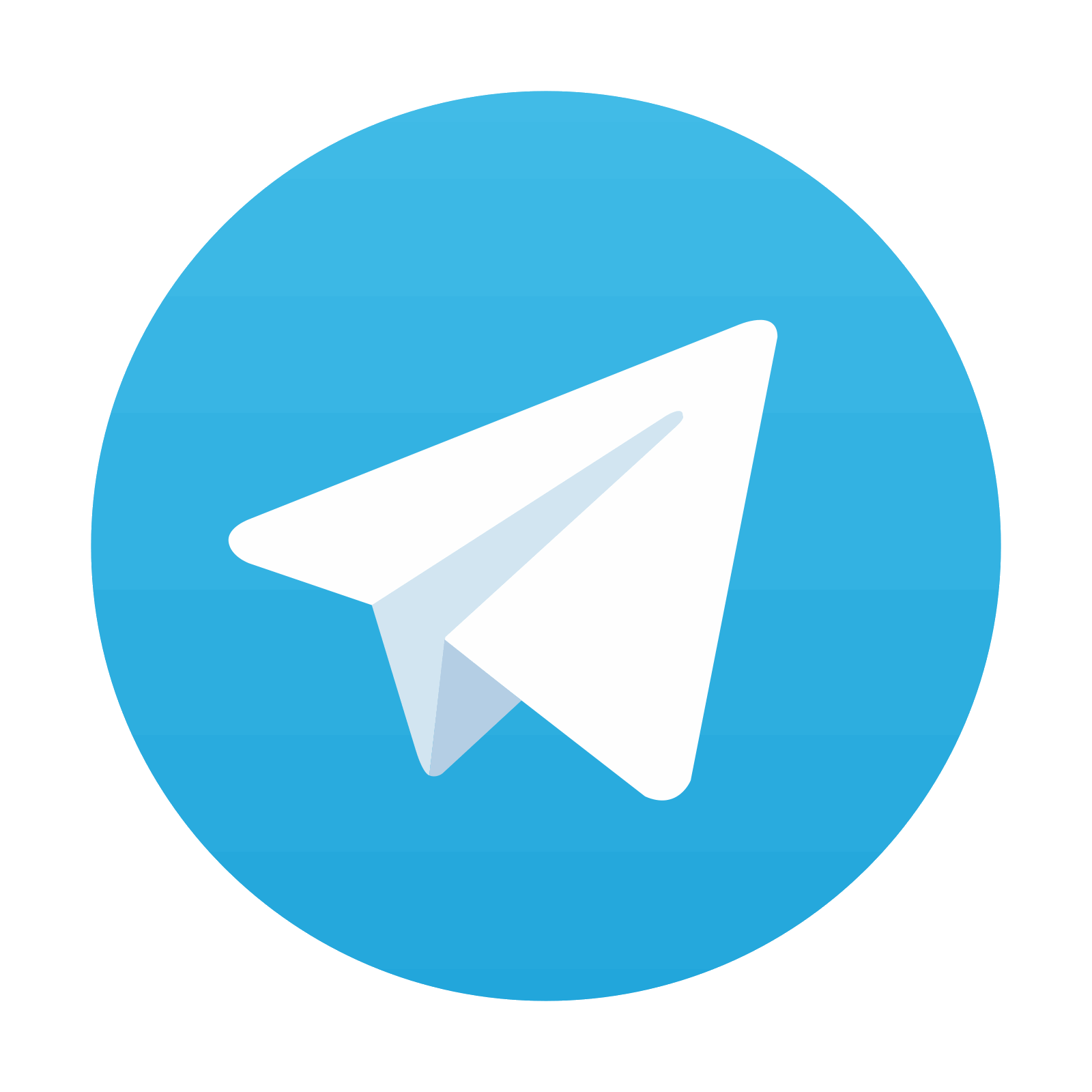
Cone beam CT with fluoroscopic precise targeting I-guide software. (a) Axial view pre-planning (b) Coronal view pre-planning (c) Reimaging after needle placement in line with the needle. (d) Fluoroscopic confirmation of projected target
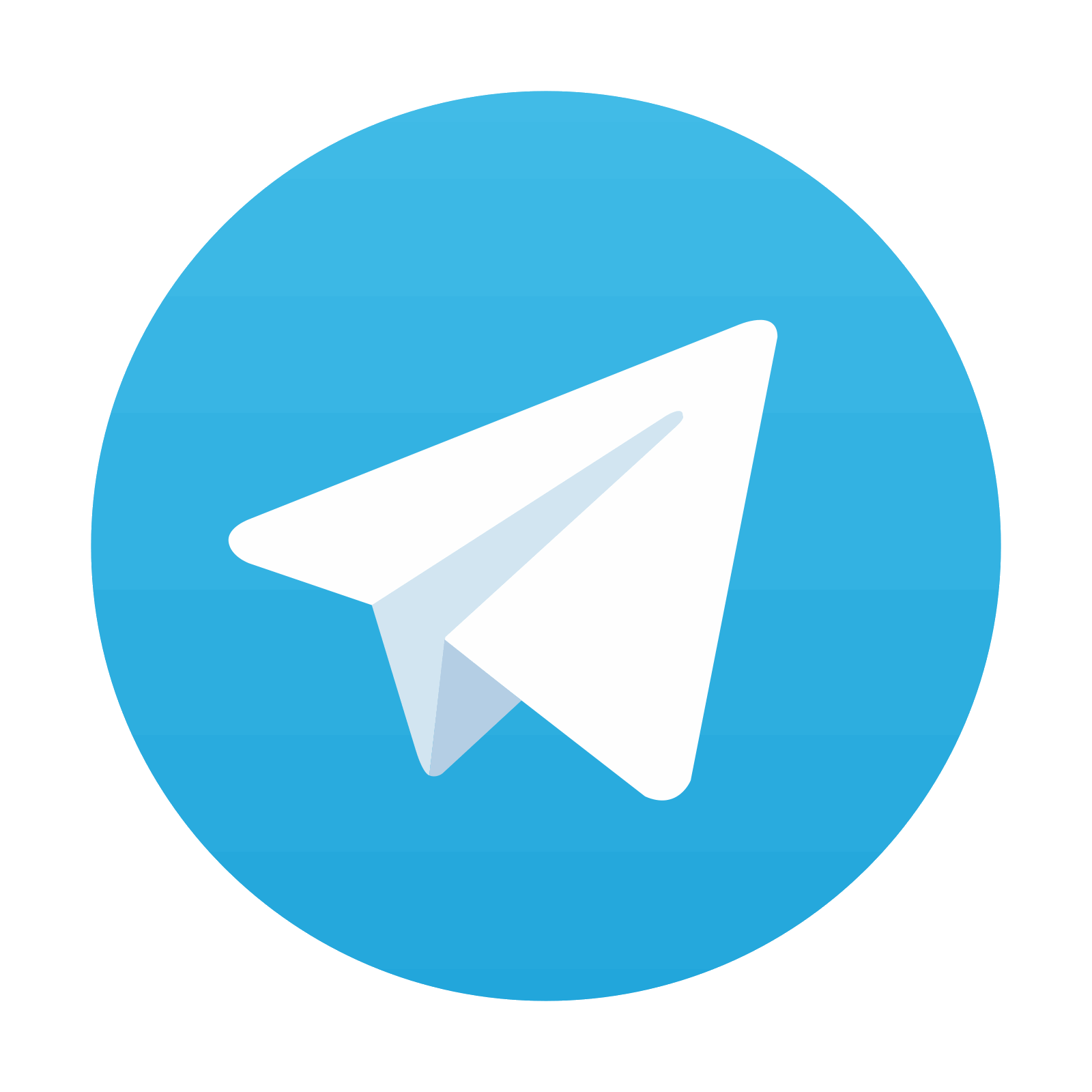
Stay updated, free articles. Join our Telegram channel
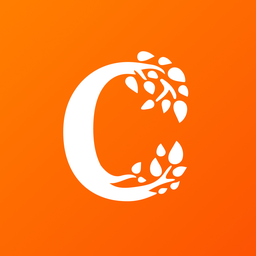
Full access? Get Clinical Tree
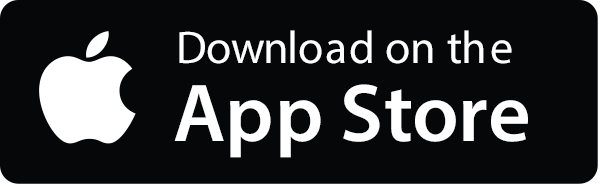
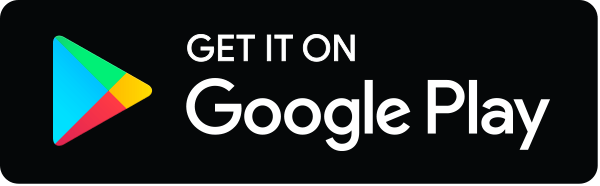
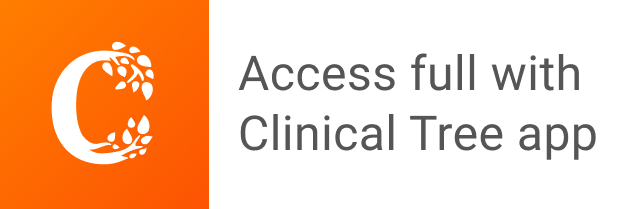