Radiation Considerations
Richard D. Nawfel MS
Stuart G. Silverman MD
Medical x-rays account for the greatest proportion (58%) of nonnatural (man-made) radiation exposure in the United States (1). With the emergence of multidetector CT, the number of multiple-acquisition CT exams, such as CT urography, has increased substantially (2, 3, 4, 5, 6 and 7). As a result, the concern for controlling the dose associated with CT has intensified. CT urography is an exam that is being used in many institutions as a replacement for conventional intravenous urography. Whereas conventional urography includes several radiographs of the abdomen and pelvis, CT urography usually consists of multiple CT acquisitions of the abdomen and pelvis (7, 8, 9 and 10). The dose from CT urography may be considerably higher than that of conventional urography. As the utilization of CT urography becomes widespread, it is important to understand the implication of radiation doses and the associated risks.
Radiation risk, CT dose quantities, factors affecting dose, and means for reducing dose are reviewed. It is hoped that radiologists will use this information when considering appropriateness of CT urography and when developing CT urography imaging protocols.
Radiation Risk
One of the most significant potential risks to patients who are examined with diagnostic CT is an increased probability of cancer due to the radiation exposure from x-rays. This risk is known as a stochastic effect. A stochastic effect is defined as one in which the probability of occurrence, rather than the severity of the effect, is proportional to the radiation dose. Stochastic effects have no threshold dose. In general, radiation doses from diagnostic x-ray imaging exams are considered to be low, and there is still a question as to whether sufficient evidence exists that establishes a risk of cancer at these low doses (11, 12). Nevertheless, in clinical practice, current radiation protection standards assume that there is no threshold dose below which the risk of cancer induction is zero. This linear nonthreshold model, substantiated in the report by the Committee on Biological Effects of Ionizing Radiation (1), is used most often in medical applications when considering radiation risk to patients. This model asserts that even the smallest radiation dose poses some finite risk (Fig. 3.1). Conversely, the linear threshold model states that there is a threshold dose below which the risk of cancer induction is zero. Although it is difficult to prove that there is no threshold dose, the linear nonthreshold model is the most conservative one describing radiation risk.
The probability of cancer induction, a stochastic effect defined previously, is the risk associated with x-ray exposure from CT. Conversely, radiation produces nonstochastic effects, or deterministic effects. These are effects in which there is usually an associated threshold. For nonstochastic effects, the severity of the effect is proportional to the radiation dose (13). Examples of nonstochastic effects are erythema and cataract induction. The dose associated with a CT exam is well below the threshold for these nonstochastic effects.
![]() Figure 3.1 Two linear radiation risk models illustrating the relationship between risk of cancer induction and radiation dose. |
The radiation risk from a single CT examination is considered small. However, compared to other diagnostic x-ray imaging procedures, the doses patients receive from a single CT exam are higher. There are several reasons for this. Since with CT the x-ray tube rotates around the patient, a CT acquisition delivers more radiation to the patient than a projection radiograph in which the x-ray tube exposes the patient from a single projection. This can lead to a substantially higher dose from CT to a specific region of the body or to critical organs compared to conventional radiography. In addition, many CT protocols, including CT urography, involve multiple acquisitions. This leads to an increased absorbed dose to the patient and thus an increased radiation risk compared to a single CT acquisition. Lastly, CT is being used in medical practice much more frequently than in the past, and CT contributes more medical radiation exposure to the population than any other imaging procedure (14, 15).
Radiation Dose Descriptors
The terms radiation dose and absorbed dose are used to describe the energy absorbed per unit mass of an irradiated material (16). The unit of radiation dose is the gray (Gy). Radiation doses from diagnostic x-ray exams may be expressed in centigray (cGy), milligray (mGy), or microgray (μmGy), depending on the magnitude. The older, conventional unit for radiation dose is the rad; 1 Gy = 100 rad. For any x-ray exam, the absorbed dose can be specified for a particular organ of interest, such as skin, thyroid, or bone marrow. Organ doses are usually estimated using radiation output data from the imaging system together with x-ray exposure factors used during the patient exam (17). Patient doses can also be measured during x-ray exams with special dosimeters.
Effective dose, previously called effective dose equivalent, is the dose quantity that accounts for nonuniform
irradiation, and it is routinely used to estimate the radiation risk for a CT exam. The effective dose corresponds to the same radiation risk of cancer and genetic effects, whether the radiation is received uniformly by the whole body or nonuni-formly, and accounts for both the distribution of radiation and the different radiosensitivities of the organs exposed (16). The unit of effective dose is the sievert (Sv). The unit that preceded the sievert was the rem; 1 Sv = 100 rem. Effective doses from single acquisition CT exams typically range from approximately 1 to 10 mSv. These doses are comparable to the annual dose from natural background radiation, approximately 3 mSv.
irradiation, and it is routinely used to estimate the radiation risk for a CT exam. The effective dose corresponds to the same radiation risk of cancer and genetic effects, whether the radiation is received uniformly by the whole body or nonuni-formly, and accounts for both the distribution of radiation and the different radiosensitivities of the organs exposed (16). The unit of effective dose is the sievert (Sv). The unit that preceded the sievert was the rem; 1 Sv = 100 rem. Effective doses from single acquisition CT exams typically range from approximately 1 to 10 mSv. These doses are comparable to the annual dose from natural background radiation, approximately 3 mSv.
Radiation dose from CT exams is estimated using a specific dose descriptor, the computed tomography dose index (CTDI). The CTDI is a measure of the integral dose along a dose profile within a tomographic section that takes into account scatter radiation from adjacent tomographic sections (18, 19, 20, 21, 22 and 23). The CTDI is estimated from measurements in dosimetry phantoms using a pencil ionization chamber or thermoluminescent dosimeters. CTDI measurements are performed during routine quality assurance dose assessment of a CT scanner. The CTDI concept has been applied to single-slice scanners, including spiral and nonspiral CT; however, with the advent of multidetector CT, the CTDI methodology is limited in estimating dose. Ionization chambers currently used do not measure completely the increased scatter radiation for beam widths associated with multidetector CT scanners. New methods for evaluating radiation dose have been proposed (24, 25
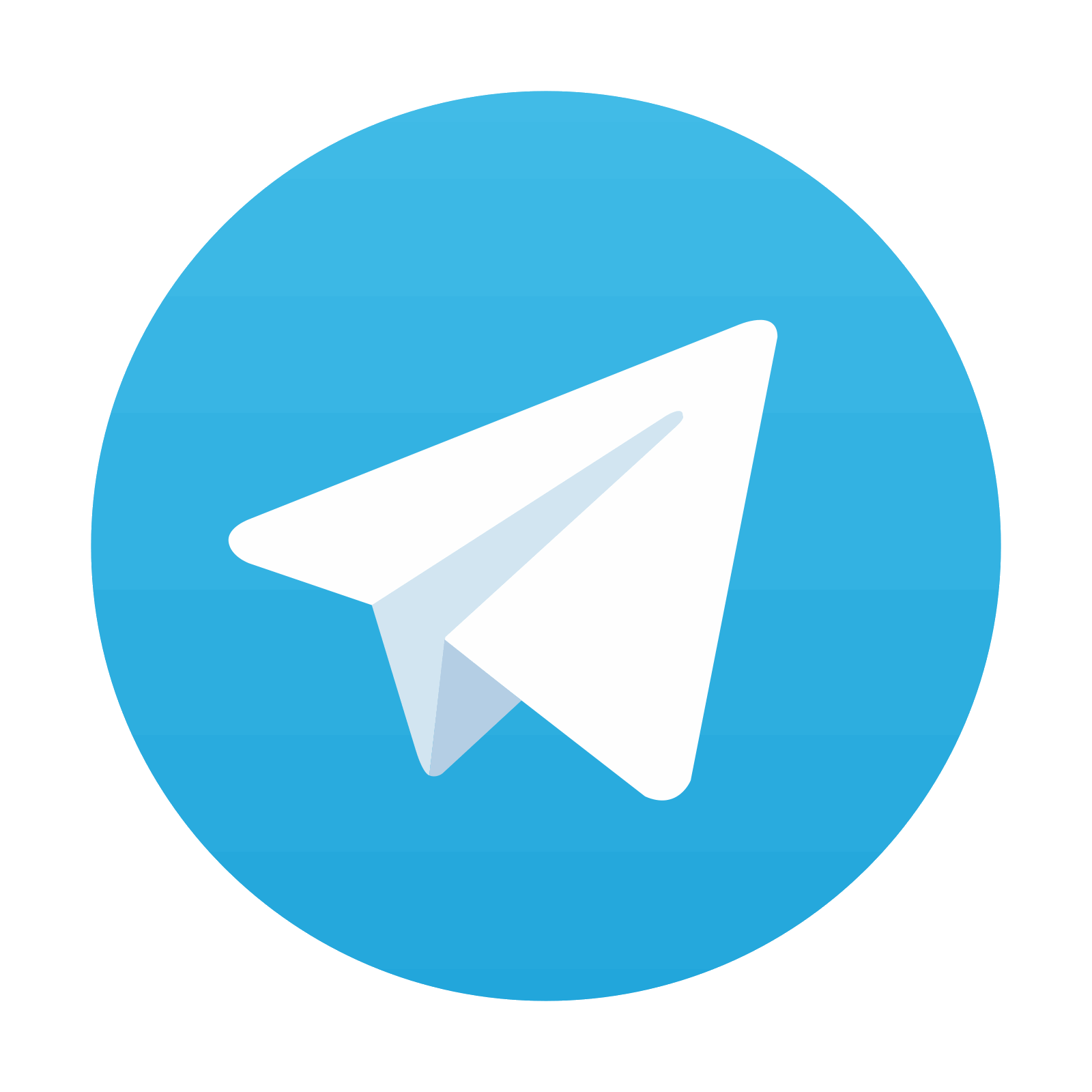
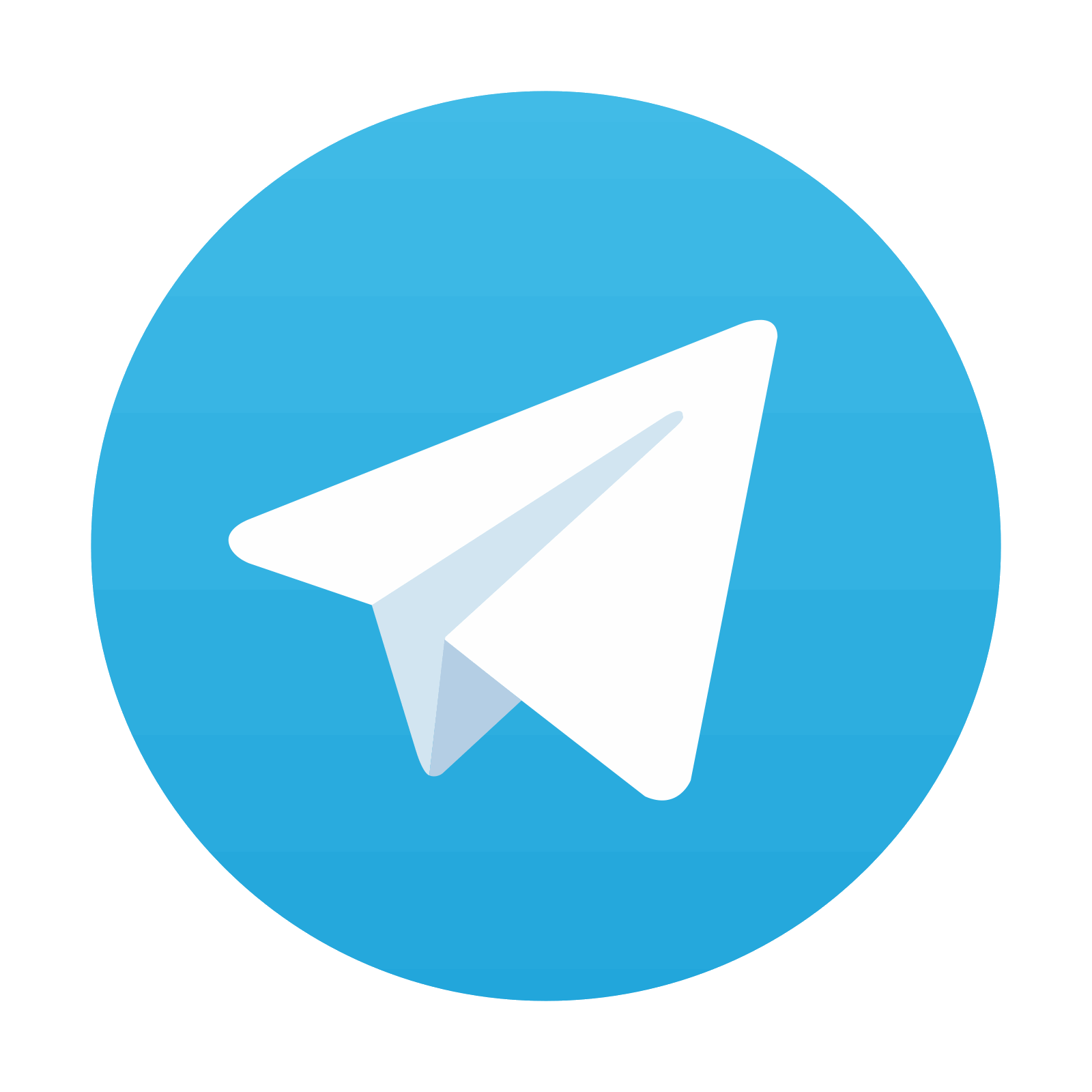
Stay updated, free articles. Join our Telegram channel
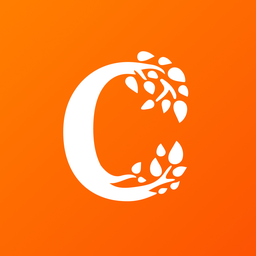
Full access? Get Clinical Tree
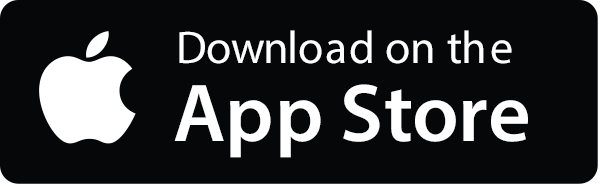
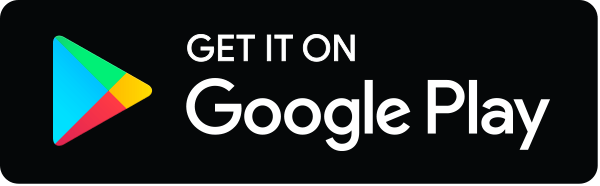