Abstract
Cholangiocytes are epithelial cells that line the network of interconnecting intrahepatic and extrahepatic bile ducts. The main physiologic function of cholangiocytes is the modification of the bile within the ductal lumen via coordinated bidirectional vectorial transport of ions, solutes, and water. These complex processes are regulated by extracellular signaling molecules, components of bile, and physical forces via various intracellular signaling pathways. The secretory and reabsorptive functions of cholangiocytes and the mechanisms by which these functions are regulated are reviewed in this chapter.
Keywords
Biliary tree, Intrahepatic and extrahepatic bile ducts, Ductal bile, Cholangiocytes, Secretion, Absorption
Cholangiocytes are epithelial cells that line the biliary tree, a complex, three-dimensional network of interconnecting intrahepatic and extrahepatic bile ducts. The main physiologic function of cholangiocytes is the modification of the bile within the ductal lumen via coordinated bidirectional vectorial transport of ions, solutes, and water. These complex processes are regulated by extracellular signaling molecules (i.e., hormones, neurotransmitters, regulatory peptides, nucleotides), components of bile (i.e., bile acids, glucose, exosomes), and physical forces (i.e., bile flow and tonicity) via various intracellular signaling pathways including cAMP and Ca 2 + signaling. In this chapter, we discuss the anatomical organization of the biliary tree, the secretory and reabsorptive functions of cholangiocytes, and the mechanisms by which these functions are regulated. The pathophysiological aspects of cholangiocytes and their role in liver diseases, collectively referred to as “cholangiopathies,” are beyond the scope of this review.
44.1
Biliary Tree
The biliary tree is a complex three-dimensional network of tubular conduits, heterogenous in size and functions, within the liver that structurally resembles a tree ( Fig. 44.1 ). In humans, the total length of the biliary tree measures approximately 1.25 miles, and the estimated volume is 20.4 cm 3 , with an internal surface area of 398 cm 2 . In addition, the surface area of the biliary tree is magnified more than fivefold by numerous microvilli on the cholangiocyte apical plasma membrane.

The biliary tree begins from the Canals of Hering, which are specialized channels located at the hepatocellular-ductular junction and lined on one side by hepatocytes and on the other side by cholangiocytes. The Canals of Hering drain into bile ductules lined only by cholangiocytes, and thus, represent the anatomic and physiologic transition from entirely hepatocyte-lined canaliculi to entirely cholangiocyte-lined ductules.
The ductules are the smallest (< 15 μm cross section) and most proximal conduits of the intrahepatic biliary tree in humans. They consecutively congregate to form interlobular ducts of 15–100 μm cross-sectional area. Two or more interlobular ducts form septal ducts (100–300 μm cross section), which then drain into area ducts (300–400 μm cross section). Area ducts converge into segmental ducts (400–800 μm cross section) and then into right and left hepatic ducts (800 μm cross section). Interlobular and septal ducts are categorized as small intrahepatic bile ducts; area, segmental, and hepatic ducts are categorized as large intrahepatic bile ducts. The intrahepatic biliary tree is connected to the extrahepatic part of the biliary tract that comprises the common hepatic duct, the cystic duct, the common bile duct, and the gallbladder (depending on species, e.g., rats, horses, zebras, camels, giraffes, elephants, and others do not have a gallbladder) ( Fig. 44.1 ).
In rodents (rats and mice), the intrahepatic biliary tree consists of small bile ducts (< 15 μm cross section) and large bile ducts (> 15 μm cross section).
In humans and rodents, small intrahepatic bile ducts in cross section are lined by 4–5 cholangiocytes; the cross section of large bile ducts may contain up to 40 cholangiocytes in humans and 8–15 cholangiocytes in rodents.
The biliary tree runs along the portal tracts adjacent to a branch of the portal vein and hepatic artery forming together an anatomical arrangement known as the portal triad ( Fig. 44.2 ). Small branches of the hepatic artery form a network of minute vessels around bile ducts, termed the peribiliary vascular plexus (PBP), which provides blood supply to the biliary tree. Blood from the PBP flows into hepatic sinusoids directly or through the portal vein branches. Despite continued use of the classic name, the portal triad also includes nerves and lymphatic vessels.

44.2
Characteristic Features of Cholangiocytes
Cholangiocytes of the biliary tree have a dual origin. Cholangiocytes of the intrahepatic bile duct are derived from bipotential progenitor cells termed hepatoblasts that give rise to both the hepatocytes and cholangiocytes. Hepatoblasts that differentiate into cholangiocyte precursors form a single layer of cells surrounding a portal vein branch, or the ductal plate, where tubulogenesis is initiated. The embryonic tubules are formed by cholangiocyte precursors on the side facing the portal tract, and by hepatoblasts that differentiate to hepatocytes on the side facing the parenchyma. When the lumen is formed, the embryonic bile duct becomes symmetrical in which hepatoblasts are replaced by cholangiocytes.
Cholangiocyte precursors express several transcription factors required for the intrahepatic bile duct development. Among them are the SRY-related high mobility group box 9 (SOX9) and 4 (SOX4) transcription factors that are considered as the earliest and most specific biliary markers. These two transcription factors are integrated in the biliary gene network and cooperate in bile duct development exerting pleiotropic effects on cell differentiation, polarization, and morphology via modulation of several signaling pathways [i.e., the transforming growth factor-β (TGF-β), Notch, Yap/Hippo and Wnt-β-catenin signaling] essential in cholangiocyte differentiation and bile duct morphogenesis.
In contrast, cholangiocytes of the extrahepatic biliary system derive from endoderm and share a common developmental origin with the pancreas and duodenum. Molecular mechanisms of the extrahepatic bile duct development remain obscure. A number of transcription factors including Pdx-1, Prox-1, HNF-6, HNF-1β, Hes-1, Sox17 are likely critical for the proper development of extrahepatic portion of the biliary tree and the gallbladder with the involvement of Notch and Hedgehog signaling. The formation of the biliary tree is completed after birth. During morphogenesis, the extrahepatic biliary tree develops before the intrahepatic biliary tree; the mechanisms by which the two anastomose are unknown.
Cholangiocytes lining different branches of the intrahepatic biliary tree are diverse in size (referred to as small and large cholangiocytes), morphology, and functions. Small cholangiocytes are 7–9 μm in diameter, flattened or cuboidal, with an inconspicuous endoplasmic reticulum and a high nucleus to cytoplasm ratio. In contrast, large cholangiocytes are 10–15 μm in diameter, columnar, with an abundant Golgi apparatus, and a low nucleus to cytoplasm ratio. These differences suggest that small cholangiocytes are less differentiated, could be progenitors of large cholangiocytes, and may have greater plasticity compared with large cholangiocytes. Indeed, small cholangiocytes in rat and mouse, which are normally mitotically dormant, proliferate in response to hormones (e.g., histamine, secretin), chemical compounds (e.g., α-naphthylisothiocyanate and carbon tetrachloride), and insults (e.g., bile duct ligation) and can acquire functional features of large cholangiocytes.
Cholangiocytes are polarized cells with apical (luminal) and basolateral plasma membrane domains. Tight junctions (zonula occludens) located near the apical plasma membrane domain seal adjacent cholangiocytes establishing and maintaining epithelial cell polarity ( Fig. 44.3 ). Two adjacent cholangiocytes are connected by gap junctions that permit direct communication between cells through the exchange of ions and small molecules.

Cholangiocytes possess a single primary cilium that extends from the apical plasma membrane and protrudes into the bile duct lumen. The primary cilium is a long, tubular organelle consisting of a membrane-bound axoneme composed of microtubules, and a centriole-derived basal body from which the axoneme emerges. The axoneme of a primary cilium has a 9 + 0 microtubule arrangement; that is, nine peripheral microtubule doublets with no central pair of microtubules, and lacks dynein arms, in contrast to that of a motile cilium which has a 9 + 2 axoneme pattern and requires motor protein dynein. Cholangiocyte primary cilia are heterogeneous in length along the biliary tree and are generally proportional to the size of the duct. In large bile ducts, the cilia are 6–8 μm in length, being approximately twice the length of that in small ducts. Basal bodies also vary in size, with diameters ranging from 0.14 to 0.26 and lengths from 0.26 to 0.48 μm in small and large bile ducts, respectively ( Fig. 44.4 ).

A significant difference between the small and large cholangiocytes exists at the levels of gene and protein expression. For example, about 4.7% of total gene expression differs between the small and large cholangiocytes in the mouse. Genes associated with cell proliferation are highly expressed in small cholangiocytes compared with large cholangiocytes. In contrast, genes associated with secretory and reabsorptive functions of biliary epithelia are overexpressed in large cholangiocytes compared with small ones. Small cholangiocytes compared with large cholangiocytes exhibit a 2- to 12-fold increase in expression of several hepatocyte transcription factors (i.e., Foxa2, HNF1a , and HNFA4 ) that regulate the expression of ntcp , aotp1 , and mrp2 , genes encoding the Na + /taurocholate cotransporter (Ntcp), the organic anion-transporting polypeptide1 (Oatp1), and the multidrug resistance-associated protein 2 (Mrp2), respectively.
Different transcriptional characteristics of the small and large cholangiocytes provide a mechanistic basis for their functional heterogeneity. For example, large but not small cholangiocytes express secretin and somatostatin receptors, the cystic fibrosis transmembrane conductance regulator (CFTR), and the Cl − -HCO − 3 exchanger, AE2, responsible for cholangiocyte secretory functions, as well as the sodium-dependent bile acid transporter, ASBT, that is involved in reabsorptive functions.
Limited information exists on the features of cholangiocytes lining extrahepatic bile ducts. In the rat, they average 16 μm in diameter, and express secretin and somatostatin (SSTR 2 ) receptors, CFTR, and AE2, suggesting they may also have functional significance associated with the modification of bile. Cholangiocyte cell lines derived from mouse extrahepatic bile ducts have recently been developed; however, no studies on these cells lines regarding the functional role of extrahepatic cholangiocytes have been reported. It has been also shown that similar to intrahepatic cholangiocytes the extrahepatic biliary epithelium in human possesses primary cilia.
44.3
Ductal Bile Formation
Modification of primary or canalicular bile secreted by hepatocytes via a series of secretory and reabsorptive processes, or ductal bile formation, is the key physiological function of cholangiocytes. In fact, about 40% of total bile in humans and about 10% in rat is a result of the secretory/reabsorptive functions of cholangiocytes. The bile formation is initiated in hepatocytes (details are depicted in Fig. 44.5 ) by active transport of organic solutes and ions followed by aquaporin (AQP)-mediated entry of water into the bile canaliculi, the minute channels (̴1 μm in diameter) bordered by the apical membranes of two adjacent hepatocytes. The vectorial transport of bile salts into the bile canaliculi provides “bile salt-dependent” canalicular bile secretion, whereas excretion of reduced glutathione, and bicarbonate drives “bile salt-independent” bile secretion. Canalicular bile subsequently enters the Canals of Hering and then flows through the biliary tree, where it is modified by secretory and reabsorptive processes that occur in large and presumably small cholangiocytes with the involvement of numerous intracellular mechanisms (details are discussed below and depicted in Fig. 44.6 ).


44.3.1
Secretory Functions of Cholangiocytes
44.3.1.1
HCO 3 − -Rich Aqueous Secretion
The signature secretory function of cholangiocytes is HCO 3 − -rich fluid secretion (i.e., ductal bile formation) into the lumen of intrahepatic bile ducts. Physiologically, ductal bile formation is a functional response of the liver to a meal. In the postprandial state, acidic pH stimulates endocrine S cells in the duodenum and jejunum to release a 27-amino-acid peptide, secretin, into the portal venous circulation. In the digestive phase, secretin regulates a number of physiological functions, including cholangiocyte HCO 3 − -rich fluid secretion, which determines the alkalinity, hydration, and pH of bile, minimizes passive cholangiocyte absorption of protonated glycine-conjugated bile acids by forming the biliary HCO 3 − umbrella, and significantly contributes to the intestinal pool of HCO 3 − required for digestion.
In the liver, only large cholangiocytes express heteromeric G protein-coupled secretin receptors (SRs) on the basolateral plasma membrane, which when activated, results in an increase in intracellular cAMP levels followed by the activation of protein kinase A (PKA) that phosphorylates and activates a cAMP-regulated Cl − channel, the CFTR , located on the apical plasma membrane. The CFTR belongs to a family of adenosine triphosphate (ATP)-binding cassette (ABC) proteins with intracellular N and C termini, and contains two domains spanning the plasma membrane six times each. These two transmembrane domains are separated by an intracellular regulatory domain containing phosphorylation sites for both PKA and protein kinase C (PKC).
Activated CFTR is a Cl − channel in an open state through which chloride ions pass out of the cell into the ductal lumen. Under basal conditions, the Cl − permeability of the cholangiocyte apical plasma membrane is low; stimulation with cAMP increases Cl − transport by 20- to 40-fold.
The current working model of cholangiocyte HCO 3 − -rich fluid secretion in humans ( Fig. 44.6 ) proposes that accumulation of Cl − in the bile duct lumen results in the activation of the apically located Na + -independent electroneutral Cl − /HCO 3 − exchanger SLC4A2/AE2 , leading to the release of HCO 3 − into the bile duct lumen in exchange for Cl − . HCO 3 − secretion is followed by osmotically driven efflux of water into the lumen of intrahepatic bile ducts primarily via water channels or AQPs. The end result is an increase in HCO 3 − -rich fluid secretion, which alkalinizes and dilutes ductal bile.
Cholangiocyte HCO 3 − secretion in rodents occurs via a Na + – HCO 3 − cotransport (NBC) carrier SLC4A4 that transports one Na + and three HCO 3 − .
Secretin-induced ductal bile secretion might be enhanced by the inhibition of the Na + /H + exchanger NHE3/SLC9A3 , which is expressed on the cholangiocyte apical plasma membrane ( Fig. 44.6 ). NHE3 stimulates absorption of fluid from the biliary lumen, thus counterbalancing the fluid secretion in resting bile duct epithelia. The PKA, activated by secretin, inhibits NHE3-mediated fluid absorption, and as a result, increases the net (secretin-induced) fluid secretion.
The secretin signal in cholangiocytes is inactivated by protein phosphatases 1 and/or 2A. These phosphatases dephosphorylate the regulatory domain of CFTR and promote conformational changes causing the occlusion of the Cl − conductance pathway, thus restoring the basal quiescent state. Thus, the ductal bile secretion stimulated by secretin is regulated at the level of CFTR by a balance between the activities of kinases (inducing activation) and phosphatases (causing inactivation) of this channel.
An alternative or additional mechanism through which CFTR contributes to the modification of bile involves Ca 2 + -activated Cl − channels . According to this model ( Fig. 44.7 ), CFTR regulates the release of ATP from cholangiocytes into the lumen of bile duct by an unknown mechanism, perhaps through ATP-permeable channels and/or exocytosis of ATP-containing vesicles. The ATP then activates apically located P2 receptors resulting in an increase in intracellular Ca 2 + and Ca 2 + -activated Cl − secretion followed by an increase in HCO 3 − and water secretion into the bile duct lumen. A recently identified Ca 2 + -activated Cl − channel, TMEM16A , on the cholangiocyte apical plasma membrane , favors this model of ductal bile secretion. The activity of TMEM16A in human, rat, and mouse cholangiocytes is increased in response to ATP, and the magnitude of ATP-stimulated TMEM16A-mediated Cl − currents is threefold greater compared with cAMP-stimulated Cl − currents mediated by CFTR. Importantly, both the small and large cholangiocytes express TMEM16A and show Ca 2 + -activated Cl − efflux in response to extracellular nucleotides, whereas only large cholangiocytes show cAMP-stimulated Cl − currents. These observations suggest that large cholangiocytes may initiate ductal bile formation via activation of both CFTR and TMEM16A, whereas small cholangiocytes may contribute to ductal bile formation via activation of TMEM16A. The contribution of CFTR and TMEM16A to cholangiocyte HCO 3 − -rich aqueous secretion may depend on species, but it is likely that TMEM16A provides the predominant mechanisms of ductal bile secretion in human and mouse cholangiocytes.

The cholangiocyte secretion of Cl − is enhanced in response to activation of a Ca 2 + -activated small conductance (SK2) K + channel located on the apical plasma membrane. In secretory epithelia, it is thought that activation of K + channels leads to membrane hyperpolarization by which the electrical driving force for continued Cl − efflux is maintained.
Thus, the mechanisms of cholangiocyte HCO 3 − -rich aqueous secretion are associated with cAMP-activated Cl − channels, CFTR, Ca 2 + -activated Cl − channels, TMEM16A, Cl − /HCO 3 exchangers, and AQPs. The apically located Na + /H + exchanger NHE3, Na + -HCO 3 − cotransport (NBC) carrier SLC4A4, and (SK2) K + channel play supportive roles in this process.
The apically located mechanisms of cholangiocyte secretion are coordinated with functions of channels, transporters, and exchangers expressed on the basolateral plasma membrane. In particular, a high concentration of intracellular HCO 3 − necessary for ductal bile secretion is maintained by coordinated functions of basolaterally located Na + – H + exchanger, NHE1/SLC9A1 , Na + – HCO 3 − cotransporter, and Na + – HCO − 3 exchanger . Cytoplasmic carbonic anhydrase, an enzyme that generates HCO 3 − from CO 2 and H 2 O, also contributes to high level of HCO 3 − .
A high concentration of intracellular Cl − is maintained by an electroneutral, bumetanide-sensitive Na + – K + – Cl − cotransporter that, under physiologic conditions, mediates transport of Na, K, and Cl ions into the cell in an electrically neutral manner with a stoichiometry of 1Na:1K:2Cl, and by Cl − -HCO − 3 exchange that provides active Cl − uptake. An increase in the apical membrane permeability to Cl − also results in the activation of two calcium-activated K + channels, SK2 and intermediate-conductance Ca 2 + -activated K + channel 1, IK-1. These channels function in a complimentary manner to support the cholangiocyte secretion via recycling of K + that was brought into the cell by the Na + -K + -2Cl − cotransporter and by Na + , K + -ATPase.
HCO − 3 secreted by cholangiocytes establishes an osmotic gradient that drives water efflux into bile duct lumen via AQPs . The AQPs are organized on the cell membrane as tetramers. The monomeric units of AQPs (26–34 kDa) contain the highly conservative NPA (asparagine-proline-alanine) motifs critical for the formation of the amphipathic pore with the diameter ranging from 1.5 to 3.4 Å, a width sufficient to allow effective passage of water molecules in single files in either direction.
The rat and mouse cholangiocytes express mRNAs for 6 AQPs and for all the 12 known AOPs, respectively. At the protein level, at least four distinct AQPs (i.e., AQP1, AQP4, AQP8, and AQP9) are expressed in mammalian cholangiocytes. Human cholangiocytes express AQP1 and AQP9.
In the basal state, AQP1 is principally expressed in rat cholangiocytes within intracellular vesicles also containing CFTR and SLC4A2/AE2. In the secretin-stimulated state, AQP1, CFTR, and AE2 are translocated to the apical plasma membrane domain facilitating HCO − 3 -driven water secretion.
The AQP1-mediated water transport across the cholangiocyte apical plasma membrane is effectively balanced by AQP4 exclusively expressed on the basolateral plasma membrane. In contrast to AQP1, AQP4 functions are not regulated by secretin suggesting that AQP4 is constitutively present in the cholangiocyte basolateral plasma membrane, or alternatively, that its functions are regulated by different signaling mechanisms.
The functional studies on isolated rat enclosed and microperfused intrahepatic bile duct units (IBDUs) in which water transport into the lumen of IBDUs in response to inward (i.e., secretory) osmotic gradients was prevented by an inhibitor of AQPs, mercuric chloride (HgCl 2 ), as well as by small interfering RNAs to AQP1, strongly supported the importance of AQP1-mediated water transport in biliary epithelia.
The role of AQP1 in ductal bile secretion in mice is controversial. Despite the fact that AQP1 is expressed in mouse small and large intrahepatic bile ducts, this water channel does not appear to be rate limiting for ductal bile formation because this process was not affected by deletion of gene encoding AQP1. Presumably, AQP8 expressed in substantial amounts in large cholangiocytes, is crucial for AQP-mediated water transport in mouse biliary epithelia.
44.3.2
Reabsorptive Functions of Cholangiocytes
Cholangiocytes contribute to the modification of bile not only by HCO 3 − -rich fluid secretion, but also by reabsorption of bile acids, glucose, amino acids, ions, water, and other biliary constituents ( Fig. 44.6 ).
44.3.2.1
Bile Acid Reabsorption
A small amount of bile acids secreted by hepatocytes is reabsorbed from the lumen of intrahepatic bile ducts and returned to hepatocytes instead of being delivered to the intestine. Unconjugated and conjugated bile acids are reabsorbed by two different mechanisms via the chole-hepatic shunt pathway. Unconjugated bile acids secreted by hepatocytes in an anionic form become protonated and passively diffuse into cholangiocytes through their apical plasma membrane. Then they are transported to the basolateral plasma membrane domain, released into the PBP, and returned to hepatocytes. In contrast, conjugated bile acids are transported across the cholangiocyte apical and basolateral plasma membrane domains by specific transporters.
The principal bile acid transporter located on the cholangiocyte apical plasma membrane is the Na + -dependent bile acid transporter ASBT/SLC10A2, a 48 kDa integral membrane protein identical to the bile acid transporter cloned from rat ileal and renal epithelial cells. ASBT/SLC10A2 is a highly efficient transporter of conjugated bile acid that mediates coupled bile acid—Na + uptake in a 2:1 ratio. An alternatively spliced form of the Asbt gene generates a truncated, 19 kDa form of ASBR, t-ASBT, located exclusively on the cholangiocyte basolateral plasma membrane presumably providing mechanisms for bile acids efflux from cholangiocytes into the peribiliary plexus. Given the toxic effects associated with intracellular accumulation of bile acids, the mechanisms of bile acids efflux from cholangiocytes via the basolateral plasma membrane are extensive and redundant. In addition to t-ASBT, these mechanisms include the multidrug resistance protein 3 (MRP3/ABCB4), MRP4, the organic anion transporting polypeptide 3 (OATP3), and the organic solute transporter OST alpha-OST beta (OSTα-OSTβ). The physiological relevance of these bile acid transporters remain to be elucidated. In general, the transcellular vectorial transport of bile acids from bile to blood may contribute to the modification of bile via regulation of its chemical composition and properties by removing some amount of bile acids and generating an osmotic pressure that facilitates AQP-mediated water absorption.
44.3.2.2
Glucose Reabsorption
Glucose enters canalicular bile at a concentration equal to plasma (i.e., 5.0–7.0 mmol/L) and is subsequently reabsorbed from bile by cholangiocytes, resulting in a low glucose concentration (i.e., < 1.0 mmol/L) when measured in bile collected from the common bile duct. The transport of glucose from bile into cholangiocytes, and from cholangiocytes into the PBP is accounted by coordinated functions of a sodium-dependent glucose transporter, SGLT1, expressed on the cholangiocyte apical plasma membrane, and by a facilitative glucose transporter, GLUT1, expressed on the basolateral plasma membrane domain. SGLT1 transports glucose into the cell against its concentration gradient utilizing the Na + -electrochemical gradient created by the Na + ,K + -ATPase. GLUT1 facilitates passive energy-independent diffusion of glucose across the cholangiocyte basolateral plasma membrane domain down its gradient ( Fig. 44.6 ).
By absorbing glucose from bile, cholangiocytes, similar to absorbing bile acids, determine the chemical composition of bile and its properties as well as establish osmotic gradients favoring water absorption. It has been shown that as the reabsorption of biliary glucose increases following d -glucose infusion into the femoral vein or portal vein, the rate of bile flow decreases. Similarly, in isolated rat liver, the rate of bile secretion decreased by one-fifth when it was perfused with a d -glucose-containing solution. In contrast, as biliary glucose absorption decreased following the administration of a competitive inhibitor of GLT1, phlorizin, bile flow increased. Moreover, direct studies on microperfused rat IBDUs showed rapid absorption of glucose from the lumen via SGLT1 followed by the AQP-mediated water absorption.
44.3.2.3
Amino Acid Reabsorption
Bile contains the amino acids glutamate, cysteine, and glycine. They are the hydrolysis products of the tripeptide, glutathione, the principal driving force for “bile salt- independent” canalicular bile secretion. Glutathione is degraded by γ-glutamyltranspeptidase, an enzyme present on the hepatocyte canalicular and cholangiocyte apical plasma membranes. Cholangiocytes absorb biliary glutamate, cysteine, and glycine by Na + -dependent and Na + -independent transport mechanisms ( Fig. 44.6 ). The functional significance of amino acid reabsorption by cholangiocytes is dual: first, absorption of glutamate, cysteine, and glycine may conserve them for glutathione resynthesis and thus may contribute to “bile salt-independent” canalicular bile secretion; and second, absorbed amino acids may generate osmotic gradients of 3–12 mOsm that favor water absorption, thereby directly involved in the modification of bile.
44.3.2.4
Bidirectional Transport of Organic Compounds
Persistent bidirectional transport (i.e., into bile and out of bile) of biliary compounds such as proteins and organic anions and cations via various mechanisms on the cholangiocyte apical and basolateral plasma membrane domains, also contributes to bile modification. Human and rat cholangiocytes express the ATP-dependent conjugate efflux pumps, MRP3/Mrp3, and MRP4/Mrp4, which transport a broad range of organic anionic substrates from bile into the systemic circulation. The multidrug resistance gene product P-glycoprotein, multidrug resistance 1 (MDR1) and Mdr1a, prevent the accumulation of organic cations in cytoplasm by excreting them back into bile. In addition, they can excrete into bile lipophilic compounds that have entered cholangiocytes from the PBP through the cholangiocyte basolateral plasma membrane.
In humans, bile contains approximately 2500 proteins that have different origins. Some of them are transported into bile by cholangiocytes. For example, epidermal growth factor (EGF) is internalized in rat cholangiocytes from the basolateral membrane via receptor-mediated endocytosis and then transported into bile by transcytosis. Polymeric IgA is secreted into bile by human cholangiocytes via a mechanism involving the IgA receptor. A number of plasma proteins, including ceruloplasmin, asialoceruloplasmin, carcinoembryonic antigen, and asialocarcinoembryonic antigen, are transported into bile presumably via anatomical communications between the fenestrated peribiliary capillary endothelium and the basolateral cholangiocyte plasma membrane. All of these transporting events influence the composition and physicochemical and physiological properties of bile flowing through the intrahepatic bile ducts.
44.4
Regulation of Ductal Bile Formation
To function in a coordinated manner and balance secretion with absorption, cholangiocyte transport systems are regulated by a variety of extracellular stimuli which we categorized into four groups: (i) stimuli that increase cholangiocyte secretion; (ii) stimuli that potentiate secretin-induced cholangiocyte secretion; (iii) stimuli that inhibit secretin-induced cholangiocyte secretion; and (iv) bile-associated stimuli.
44.4.1
Stimuli That Increase Cholangiocyte Secretion
Despite the fact that secretin is considered the principal regulator of cholangiocyte HCO 3 − -rich fluid secretion, a number of other hormones and regulatory peptides may increase ductal bile secretion. Among them are bombesin, vasoactive intestinal peptide (VIP), and corticosteroids.
Bombesin, also known as gastrin-releasing peptide, stimulates cholangiocyte HCO 3 − rich fluid secretion in vitro and in vivo directly or indirectly by inducing the release of other secretagogues. Bombesin-stimulated cholangiocyte secretion is due to increased Cl − /HCO 3 − exchanger activity, coupled to apical Cl − channels, and is counterbalanced by electrogenic Na + -HCO 3 − cotransporter and K + channels on the basolateral plasma membrane.
The VIP stimulates cholangiocyte HCO 3 − -rich fluid secretion in human and rat. In rats, VIP was reported to be a more potent stimulus of biliary secretion than either secretin or bombesin.
Corticosteroids (e.g., dexamethasone) or drugs of the corticosteroid type (e.g., budesonide) increased biliary HCO 3 − -rich fluid secretion in vivo and in IBDUs isolated from rat livers via upregulation of two exchangers critical for ductal bile modification, namely the apical Cl − /HCO 3 − exchanger and basolateral Na + /H + -exchanger (NHE1 isoform).
44.4.2
Potentiation of Secretin-Stimulated Cholangiocyte Secretion
Acetylcholine and adrenergic agonists belong to stimuli that do not influence basal cholangiocyte secretion, but potentiate secretin-stimulated cholangiocyte HCO 3 − -rich fluid secretion.
Acetylcholine (ACh) potentiates secretin-stimulated HCO 3 − -rich fluid secretion via activation of muscarinic M1 and M3 receptors that are expressed on the cholangiocyte basolateral plasma membrane. In isolated bivascularly perfused rat liver, arterial infusion of ACh accentuates secretin-stimulated HCO 3 − -rich fluid secretion. In contrast, a decrease in secretin-stimulated HCO 3 − -rich fluid secretion was observed in cholangiocytes isolated from the liver of bile duct-ligated rats with total vagotomy; this further supports the role of parasympathetic innervation and its neurotransmitter, ACh, in the potentiation of secretin-stimulated HCO 3 − -rich fluid secretion.
Adrenergic agonists (e.g., phenylephrine) potentiate secretin-stimulated HCO 3 − -rich fluid secretion in bile duct ligated (BDL) rats in vivo and in an in vitro model of enclosed IBDUs. The effects of phenylephrine were abolished by benoxanthian hydrochloride, α1-adrenergic antagonist, suggesting that the adrenergic agonists potentiate ductal bile modification via activation of adrenoreceptors. In contrast, degeneration of adrenergic innervation with 6-hydroxidopamine in BDL rats resulted in a decrease in secretin-stimulated choleresis.
44.4.3
Inhibition of Basal and Secretin-Stimulated Cholangiocyte Secretion
The secretin-stimulated cholangiocyte secretion may be terminated or inhibited by several regulatory stimuli such as somatostatin, gastrin, dopaminergic agonists, alpha2-adrenergic receptor agonists, endothelin (ET), and gamma-aminobutyric acid (GABA).
Somatostatin, a cyclic tetradecapeptide produced by a variety of tissues, including but not limited to neurons in the central and peripheral nervous system and neuroendocrine cells of the gastrointestinal tract, inhibits both basal and secretin-stimulated ductal fluid secretion in human, dog, rat, and mouse. A wide array of physiological effects mediated by somatostatin involve five somatostatin receptor subtypes (sst1-sst5). Four of them, sst1-sst4, are expressed on the cholangiocyte `basolateral plasma membrane domain in rat and mouse. In addition, enhanced ductal fluid reabsorption was suggested as one of the major mechanism of somatostatin-induced anticholeresis in mice and dogs. Indeed, in IBDUs from normal mice, somatostatin and L-779976, a somatostatin analog specific for sst2, not only inhibited secretin-stimulated ductal fluid secretion, but also directly induced ductal fluid absorption; in sst2 knockout mice, these effects were diminished. Thus, somatostatin may regulate ductal bile formation by two mechanisms: (i) inhibiting basal and secretin-stimulated cholangiocyte HCO 3 − -rich fluid secretion and (ii) directly stimulating cholangiocyte fluid absorption.
Gastrin , a linear peptide produced and secreted by G cells in the duodenum and gastric antrum in response to a variety of stimuli, effectively inhibits secretin-stimulated cholangiocyte secretion in BDL via activation of the cholecystokinin-B/gastrin receptors.
Dopaminergic agonists (e.g., quinelorane) inhibit secretin-stimulated cholangiocyte secretion in BDL rats via activation of dopaminergic receptors, D2, expressed on the cholangiocyte basolateral plasma membrane.
Alpha2-adrenergic receptor agonists (e.g., UK14,304) inhibit secretin-stimulated ductal bile secretion in BDL rats in contrast to the activation of alpha1-adrenergic receptors that potentiate secretin-stimulated HCO 3 − -rich fluid secretion. Similarly, degeneration of adrenergic innervation with 6-hydroxydopamine in BDL rats was found to result in a decrease in cholangiocyte cAMP levels and secretin-stimulated choleresis.
Endothelin , a 21-amino-acid polypeptide with multifunctional properties, inhibits secretin-stimulated cholangiocyte secretion by interacting with ET A receptors in BDL rats.
Gamma-aminobutyric acid (GABA) , a principle inhibitory neurotransmitter in the nervous system of vertebrates, inhibits secretin-induced Cl − efflux in BDL rats via the activation of GABAA and GABAB receptors.
44.4.4
Bile-Associated Stimuli
The composition, osmolarity, and flow rates of bile transported through the biliary tree vary over time and space. These properties of bile significantly affect cholangiocyte secretory and absorptive functions via a number of channels and receptors expressed on the cholangiocyte apical plasma membrane and primary cilia.
Bile acids influence ductal bile secretion via several mechanisms. As mentioned earlier, unconjugated bile acids absorbed by cholangiocytes stimulate HCO 3 − -rich choleresis. For example, ursodeoxycholic acid (UDCA) induces Cl − efflux via Ca 2 + -activated Cl − channels expressed on the cholangiocyte apical plasma membrane either directly or more likely through CFTR-dependent ATP secretion. As a result, HCO 3 − -rich fluid secretion is increased.
Unconjugated and conjugated bile acids may regulate ductal bile secretion via the G protein-coupled receptor, TGR5, expressed on the cholangiocyte apical plasma membrane and cilia. Based on experiments conducted with isolated gallbladder epithelial cells, TGR5 colocalizes with CFTR, and its stimulation results in the activation of CFTR and increase in Cl − secretion. The involvement of TGR5 in bile acid-induced ductal bile secretion is supported by observations that TGR5 knockout mice have reduced bile flow compared with wild-type littermates, and that in rats and mice intravenous, intraperitoneal, and oral administration of the TGR5 agonist, INT-777, increased bile flow. The working hypothesis is that TGR5 function as a sensor coupling bile composition to cholangiocyte Cl − , HCO 3 − , and fluid secretion.
ATP and other nucleotides present in bile activate P2Y receptors located on the cholangiocyte apical plasma membrane causing a rapid and substantial increase in membrane Cl − permeability, favoring efflux of Cl − from the cell into the ductal lumen through Ca 2 + -activated Cl − channel, TMEM16A. In rat IBDUs, activation of the apical plasma membrane P2Y receptors results in an increase in biliary HCO 3 − secretion, thus suggesting the importance of this mechanism in ductal bile modification.
Bile flow exerts mechanical forces on the cholangiocyte apical plasma membrane and cilia and induces an increase in secretion of Cl − and HCO 3 − in the lumen of intrahepatic bile ducts. The cholangiocyte senses bile flow via the primary cilium as well as by yet unknown mechanisms associated with ATP release and activation of P2Y receptors in response to mechanical forces applied to the apical plasma membrane.
An additional mechanism through which mechanical forces generated by bile flow regulate ductal bile formation is the epithelial Na + channel (ENaC) expressed on the cholangiocyte apical plasma membrane. This channel is involved in coordination of cholangiocyte secretion and absorption that is achieved by ATP-dependent regulation of the Ca 2 + -activated Cl − channel, TMEM16A, and the ENaC. Cholangiocyte secretion occurs when ATP that is released into the bile duct limen in response to bile flow activates TMEM16A and inhibits ENaC. A decrease in liminal ATP causes inactivation of TMEM16A and activation of ENaC, resulting in net fluid absorption.
Bile osmolality influences the activity of TRPV4, a Ca 2 + channel expressed on the cholangiocyte apical plasma membrane and cilia. TRPV4 is activated by extracellular hypotonicity and inhibited by extracellular hypertonicity. Exposure of cholangiocytes to hypotonicity induced an increase in intracellular Ca 2 + , cilia-dependent ATP release, and HCO 3 − secretion, suggesting an important role of cilia-associated TRPV4 in ductal bile formation. This observation was supported by in vivo experiments, in which a retrograde infusion of 4αPDD, an agonist to TRPV4, into rat intrahepatic bile ducts induced ductal bile secretion; this effect was abolished by Gd 3 + , a TRP4 channel inhibitor.
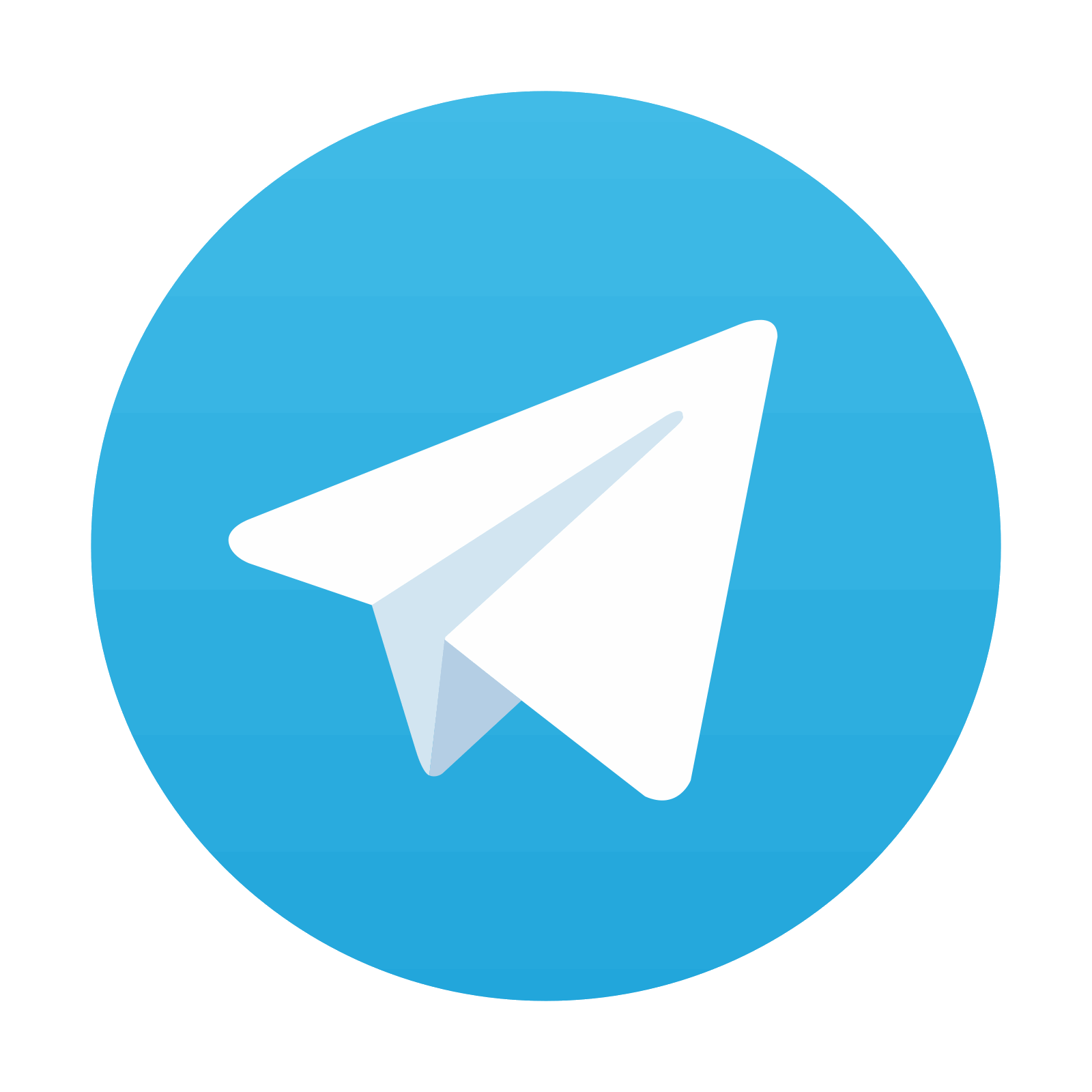
Stay updated, free articles. Join our Telegram channel
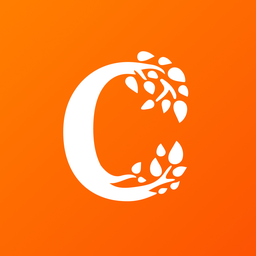
Full access? Get Clinical Tree
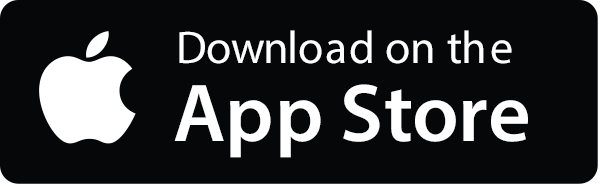
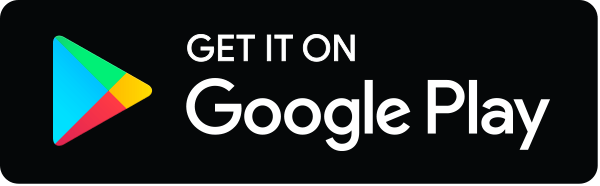